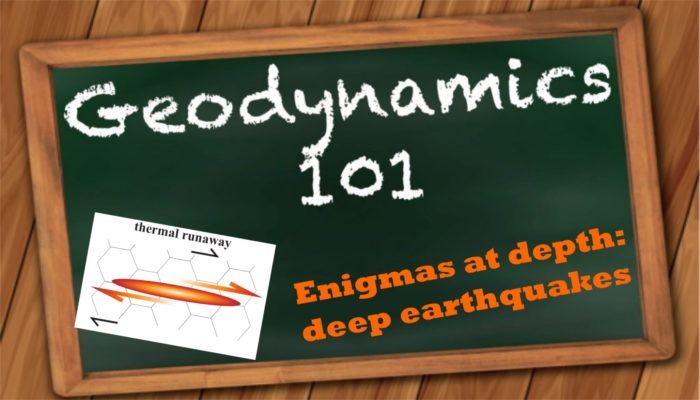
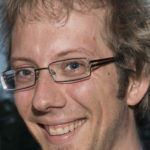
Dr. Marcel Thielmann.
The Geodynamics 101 series serves to showcase the diversity of research topics and/or methods in the geodynamics community in an understandable manner. In this week’s Geodynamics 101 post, Marcel Thielmann, Senior Researcher at the University of Bayreuth, discusses the possible mechanisms behind the ductile deformation at great depths that causes deep earthquakes.
Earthquakes are one of the expressions of plate tectonics that everybody seems to be familiar with. When I started studying geophysics, people used to ask me what exactly I was studying. As soon as I mentioned earthquakes, I usually got a knowing nod and no further questions were asked (the same goes for volcanoes, but that’s a topic for another day).
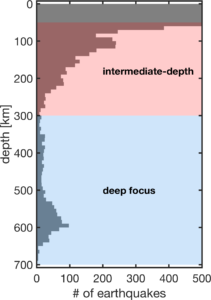
Figure 1: Global hypocentre distribution of earthquakes with a magnitude Mw>5 in the ISC catalogue for the interval 1960-2013. The x-axis has been truncated for better visibility.
Most earthquakes occur at the boundaries of tectonic plates, where rock breaks due to the forces originating from the plates’ relative movement. In 1928, Kiyoo Wadati discovered earthquakes that occurred at depths larger than 60 km, which were previously thought to be impossible. Today, we know that these earthquakes are not that extraordinary: about one out of four earthquakes observed on Earth occurs at depths larger than 60 km. At this depth, the pressure inside the Earth reaches values of about 3 GPa and more. Laboratory experiments have shown that at this pressure, rocks do not deform by breaking, but rather by ductile creep, like putty. This kind of deformation should not produce any earthquakes. So, 90 years after their discovery, the question still remains: What causes deep earthquakes?
How do rocks fail at these high pressures?
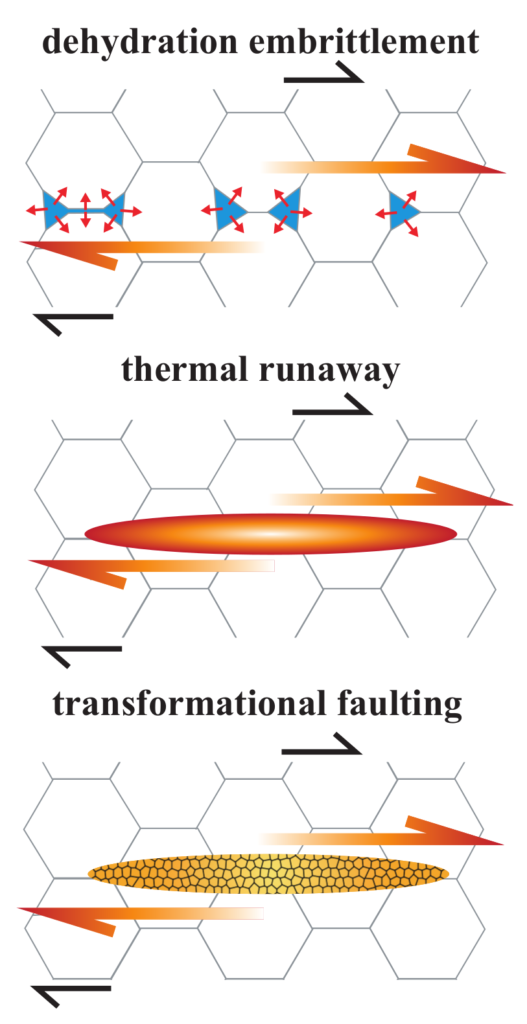
Figure 2: Schematic view of the three proposed ductile failure mechanisms.
As rocks get transported to larger depths, the minerals making them up can experience phase transformations. Due to these transformations, two things may happen: (1) Previously stored water in the minerals is released. This release may trigger earthquakes due to the released water acting against the pressure of the surrounding rock in a mechanism called dehydration embrittlement (Green and Houston, 1995; Frohlich, 2006). (2) The phase transition renders a fine-grained rock that is easier to deform. If enough of this weak material is produced, rock failure occurs in a process called transformational faulting (Green and Houston,1995; Ferrand et al.,2017). Besides these two mechanisms, a third one called thermal runaway has been thrown into the ring (Hobbs et al., 1986; Ogawa, 1987). This mechanism is a result of shear heating, which describes the generation of heat inside a deforming rock. If heat generation is faster than its transport, temperatures inside the rock will continue to increase and ultimately result in its destabilization, thus causing an earthquake.
The Wind River earthquake
While most of the observed deep earthquakes occur in subduction zones, where one tectonic plate descends beneath another, there are some that occur far from them. One such earthquake hit the Wind River range in Wyoming with a magnitude of MW 4.7 in 2013 (Frohlich et al., 2015; Craig and Heyburn, 2015). This earthquake is not only enigmatic due to its depth of 75 km (making it the second deepest earthquake in such a stable continental region), but also because the Wind River area is considered to be “seismically quiet”. The location of the earthquake is far away from any plate boundary, with the closest tectonic feature being the Yellowstone supervolcano more than 200 km away. Since it occurred, the cause of this earthquake has been a matter of debate, with some scientists preferring a purely brittle origin (Craig and Heyburn, 2015), while others argue for a ductile mechanism (Prieto et al., 2017).
Dehydration embrittlement seems to be an unlikely candidate, since the earthquake is located far away from any subduction zone. How could fluids get down to those depths if not by subduction? Transformational faulting also seems to be unlikely, since this would require a phase transition to take place. The Wind River earthquake occurred in the continental mantle lithosphere, where we would not expect any major phase transitions. Thermal runaway may be a candidate, but studies have shown that very high stresses are required to make this mechanism work, stresses that are very hard to achieve in the Earth.
However, there may be a way out: grain size assisted thermal runaway. Oh no, yet another one you might think. But fear not, this mechanism is essentially the same as the “classical” thermal runaway, just with the effect of small grains included. The consequences of this effect are by no means small however, as it significantly reduces the stresses required for thermal runaway. Indeed, numerical models of this process at the conditions of the Wind River earthquake indicate that it may indeed be a viable mechanism to have generated this earthquake (Thielmann, 2018). However, these models also show that rock deformation has to be sufficiently fast (about 100 times faster than what is commonly assumed) in order to allow for earthquake generation.
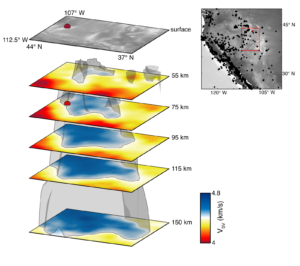
Figure 3: Location and mantle structure of the 2013 Wind River earthquake. Inset: Location within the north-western US. Black points represent earthquakes larger than Mw 4.5 from the NEIC catalogue. The red circle indicates the location of the Wind River earthquake. The red box denotes the region of the main figure. Main Figure: Seismic velocity structure and hypocentre location. Tomographic data is taken from Shen et al. (2013). Colours denote seismic velocities, with blue colours indicating faster and red colours slower velocities. Fast seismic velocities are commonly associated with colder and denser material. The red spheres denote the location of the hypo- and epicentre. The grey isosurface at 4.4 km/s delineates the dense body extending to larger depths.
So now we have shifted the question from “How could fluids get down to those depths if not by subduction?” to “How could we deform that fast at those depths?” Here, seismology may come to the rescue: tomographic models of the north-western United States show that the Wind River earthquake lies directly at the transition between two regions with strongly varying seismic wave speeds (Shen et al., 2013; Wang et al., 2016). Fast wave speeds are commonly seen as an indicator for cold material, while slow wave speeds indicate warm material. 3D seismic tomographies such as the one from Shen et al. (2013) show that the 2013 Wind River earthquake occurred in a region where the continental lithosphere may be detaching in the form of a drip (Wang et al., 2016). In such tectonic environments, deformation rates may reach the values needed to initiate grain size assisted thermal runaway (Lorinczi and Houseman, 2009).
Does this now answer all questions we have on the Wind River earthquake and deep earthquakes in general? Certainly not. The example given above was just a single instance of where the combined information from seismology, laboratory experiments and numerical modelling may help us find an answer. We still have to keep in mind G.E.P. Box’s famous expression „Essentially, all models are wrong, but some are useful“. It is certain that deep earthquakes contain a wealth of information that remains to be unlocked. The following quote by Heidi Houston (2015) points the way:
Integration of seismological, laboratory, and modelling effort is needed to bridge the stubborn gap between source properties, which are extracted under strong assumptions and possess substantial intrinsic variability, and physical mechanisms of rupture generation, which are as yet neither well understood nor well constrained. (H. Houston)
References Craig, T. J., and R. Heyburn (2015), An enigmatic earthquake in the continental mantle lithosphere of stable North America, Earth Plan. Sc. Lett., 425, 12–23, doi:10.1016/j.epsl.2015.05.048. Ferrand, T., N. Hilairet, S. Incel, D. Deldicque, L. Labrousse, J. Gasc, J. Renner, Y. Wang, H. W. Green II, and A. Schubnel (2017), Dehydration-driven stress transfer triggers intermediate-depth earthquakes, Nat. Commun., 8, 15247, doi:10.1038/ncomms15247. Frohlich, C. (2006), Deep Earthquakes, Cambridge University Press. Frohlich, C., W. Gan, and R. B. Herrmann (2015), Two Deep Earthquakes in Wyoming, Seismological Research Letters, 86(3), 810–818, doi:10.1785/0220140197. Green, H. W., and H. Houston (1995), The Mechanics of Deep Earthquakes, Annu. Rev. Earth. Planet. Sci., 23, 169–213. Hobbs, B. E., A. Ord, and C. Teyssier (1986), Earthquakes in the Ductile Regime, Pure Appl. Geophys., 124(1-2), 309–336. Houston, H. (2015), 4.13 - Deep Earthquakes, in Treatise on Geophysics (Second Edition), edited by G. Schubert, pp. 329–354, Elsevier, Oxford. Lorinczi, P., and G. A. Houseman (2009), Lithospheric gravitational instability beneath the Southeast Carpathians, Tectonophysics, 474, 322–336, doi:10.1016/j.tecto.2008.05.024. Ogawa, M. (1987), Shear instability in a viscoelastic material as the cause of deep focus earthquakes, J. Geophys. Res., 92, 13,801–13,810. Prieto, G. A., B. Froment, C. Yu, P. Poli, and R. Abercrombie (2017), Earthquake rupture below the brittle-ductile transition in continental lithospheric mantle, Sci. Adv., 3(3), e1602642, doi:10.1126/sciadv.1602642. Shen, W., M. H. Ritzwoller, and V. Schulte Pelkum (2013), A 3‐D model of the crust and uppermost mantle beneath the Central and Western US by joint inversion of receiver functions and surface wave dispersion, J. Geophys. Res., 118(1), 262–276, doi:10.1029/2012JB009602. Thielmann, M. (2018), Grain size assisted thermal runaway as a nucleation mechanism for continental mantle earthquakes: Impact of complex rheologies, Tectonophysics, 746, 611–623, doi:10.1016/j.tecto.2017.08.038. Wang, X., D. Zhao, and J. Li (2016), The 2013 Wyoming upper mantle earthquakes: Tomography and tectonic implications, J. Geophys. Res., 121(9), 6797–6808, doi:10.1002/2016JB013118.