Large subduction earthquakes cause perturbation of the subduction system, whose response depends on its rheological characteristics. The evolution of the subduction system from earthquake to earthquake is defined as subduction earthquake cycle (SEC), and is characterised by three main processes that operate over decades/centuries: postseismic afterslip, viscoelastic mantle relaxation, and fault re ...[Read More]
Geomythology. Chimera: the lion, the goat, the snake and the flaming gas vents
Once upon a time in Lycia there was a land of fire, which burned so bright the light was visible by sailors navigating on the Mare Lycium, who were using the fires as a natural lighthouse. “Mount Chimaera in the country of Phaselis is on fire, and indeed burns with a flame that does not die by day or night”; those were the words of Pliny the Elder in his Naturalis Historia (2.110). Ancient Lycia i ...[Read More]
Geotandem: The Tectonics (or lack thereof) of Mars
Welcome to the first of its kind, the Geotandem 01! A collaborative series between EGU divisions. Interdisciplinarity is intrinsic to Geosciences, so we want to showcase how researchers approach the same topics from different but also complementing perspectives. In each edition, we will bring you a high-interest topic for the community seen from the eyes of diverse disciplines. Today, the Tectonic ...[Read More]
TS Must-Read – Mancktelow (2008): Tectonic pressure: Theoretical concepts and modelled examples
Neil Mancktelow published this Must-Read paper on the concept of “tectonic pressure” in 2008. The paper reviews previous work and theoretical concepts published on this fundamental topic. Additionally, numerical models that estimate the magnitude of tectonic pressure variations are presented for several realistic natural structures, such as folds, boudins, and inclusions. The premise of tectonic p ...[Read More]
TS Must-Read – Bürgmann and Dresen (2008): Rheology of the Lower Crust and Upper Mantle: Evidence from Rock Mechanics, Geodesy and Field Observations
In 2008 Roland Bürgmann and Georg Dresen published their Must-Read paper on the rheology of the lower crust and upper mantle, based on findings from the lab, the field and space. As stated in the introduction, “rheology is the study of the flow and deformation of all forms of matter,” and as such the rheology of the Earth’s lower crust and upper mantle is closely linked to the evolution and deform ...[Read More]
TS Must-Read – Hudec and Jackson (2007) Terra infirma: Understanding salt tectonics
The TS Must-Read paper by Hudec and Jackson (2007) provides a combination of analogue models and natural cases to describe, in a review paper, salt flow mechanisms, diapir growth, and the ways these processes interact with regional deformation, in compression and extensional tectonics. Salt is mechanically weak and can flow like a fluid under gravitation, displacement, and thermal lo ...[Read More]
TS Must-Read – Bond et al. (2007) What do you think this is? “Conceptual uncertainty” in geoscience interpretation
The Must-Read paper by Bond et al. (2007) is a truly original piece of work, focusing on the conceptual uncertainty that occurs when earth scientists carry out a geological interpretation on data with limited resolution and/or spatial coverage. In this example it is about an interpretation of seismic reflection data (Fig. 1), but it can be applied to other situations as well. Conceptual uncertaint ...[Read More]
Geomythology. Devils tower: born from hell?
Geomythology. Devils tower: born from hell? Following the previous two posts (Giant’s Causeway – the mythical stone way; Columnar Basalts and why Hexagons are nature’s favourite shape) it is clear how columnar joints are quite common around the globe, and they create such a marvelous natural environment that inspired amazing myths and legends: the Devils Tower myth is one of them. Devils Tow ...[Read More]
Remembering Janos Urai – A master of all scales
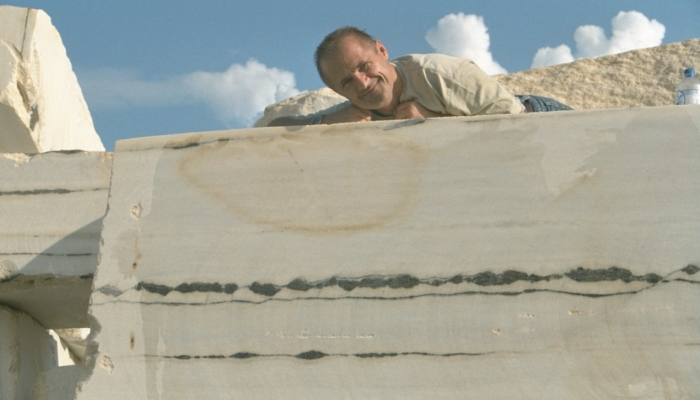
As many will know, on Sunday, 28 May 2023, one of the most prominent, energetic and valued members of the Tectonics and Structural Geology community, Emeritus Professor Janos Urai, was lost in a tragic climbing accident in Belgium. Here we remember Janos for the amazing scientist that he was and for the huge legacy that he has left, in the form of his own transformative contributions and the inspi ...[Read More]
Exploring Submap: Subduction Zones Data Mapping and Analysis in just a few clicks
Subduction zones are areas where one tectonic plate dives beneath another one and sinks into the Earth’s mantle, creating powerful earthquakes and volcanic eruptions. Understanding the intricate details of subduction zones can be a daunting task, and retrieving the necessary data often feels like an uphill battle. But fear not! The Geosciences Montpellier Laboratory in France has come to the rescu ...[Read More]