This week one of our editor’s, Aisling Dunn, brings back the book reviews, talking through her thoughts on her most recent read and why she recommends you all read it too. There’s no doubt about it – I am a self-confessed book lover. Whether its fantasy stories about imagined worlds, classic tales set in 19th century England, travel writing, historical non-fiction, biographies, there are ver ...[Read More]
Watch and learn!
Too envious to watch Netflix’s Selling Sunset? Gone through the top 5 geodynamic movies? Lock-down vacation too boring for words? In need of useful procrastination? Search no longer! We have compiled an extensive list of online webinars and speaker series that were already active pre-Corona or saw the daylight during the pandemic to stay in touch with our colleagues both abroad and next door ...[Read More]
What controls Victoria microplate rotation in the East African Rift?
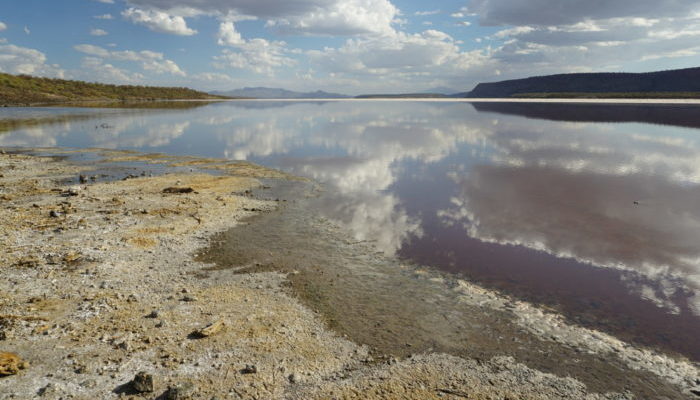
This week in News & Views, Anne Glerum, postdoc at GFZ Potsdam, discusses how her numerical models support a lithosphere-driven mechanism for the rotation of large continental microplates, like Victoria in the East African Rift System. The East African Rift System (EARS) is a newly forming divergent boundary between the Nubian and Somalian plates (Fig. 1). The plate boundary system includes se ...[Read More]
Starting to teach? Here are 3 tips!
Starting to teach a course at a university can be an exciting but daunting experience. In today’s post Menno Fraters shares his experiences of starting to teach a class of 100+ students during the challenging time of the COVID-19 pandemic. He provides three quick tips for when you start teaching, that may be useful for teaching in academics. During your time as a PhD student, you may have al ...[Read More]