If the title and image didn’t tip you off: the EGU Geodynamics blog is celebrating its first anniversary! Almost exactly 1 year ago (okay, so it’s one year and one day, because I wanted to stick to the Wednesday upload schedule), the EGU GD blog was launched! Yay! Applause! Good thing we’re not insanely vain about or proud of this and going to milk this event with a blog post. Oh ...[Read More]
Magma oceans
The Geodynamics 101 series serves to showcase the diversity of research topics and methods in the geodynamics community in an understandable manner. We welcome all researchers – PhD students to professors – to introduce their area of expertise in a lighthearted, entertaining manner and touch upon some of the outstanding questions and problems related to their fields. For our latest ‘Geodynamics 10 ...[Read More]
Remarkable Regions – The Kenya Rift
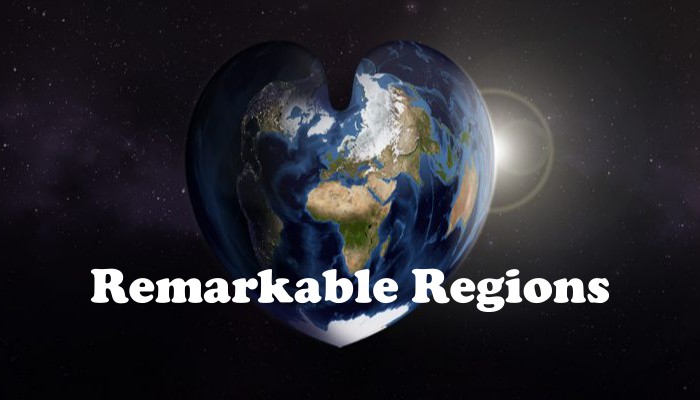
Every 8 weeks we turn our attention to a Remarkable Region that deserves a spot in the scientific limelight. After looking at several convergent plate boundaries, this week the focus lies on part of a nascent divergent plate boundary: the Kenya Rift. The post is by postdoctoral researcher Anne Glerum of GFZ Potsdam. Of course an active continental rift is worthy of the title “Remarkable Region”. A ...[Read More]
The art of the 15-minute talk
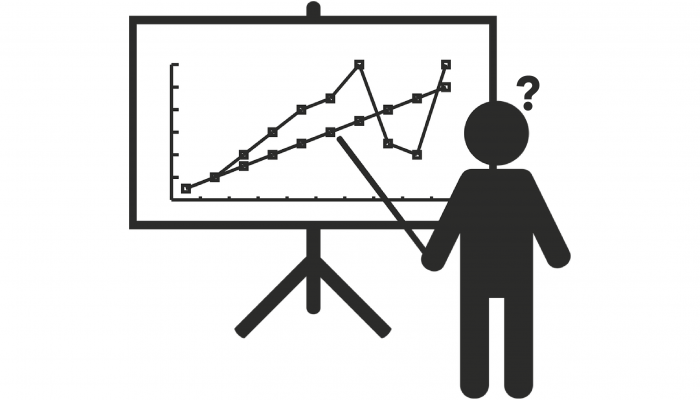
Credit: Pixabay
We’ve all attended conferences with those dreaded 15-minute talks and we have no problem picking out which talks were amazing and which talks were abysmal. However, when it comes to our own talks, it’s hard to judge them, find out how they can be improved or break away from long-established habits (such as our layout or talking pace). This week, Matthew Herman, postdoc at the Tectonoph ...[Read More]