Subduction zones host one of the most complex and fascinating tectonic systems on the planet. Numerical models by Király and colleagues recently published in Earth and Planetary Science Letters reveal that the strength of the toroidal flow depends on the mantle viscosity and the magnitude of the slab pull force while the characteristic size of the toroidal cells mainly depends on the size of the c ...[Read More]
Going with the toroidal mantle flow
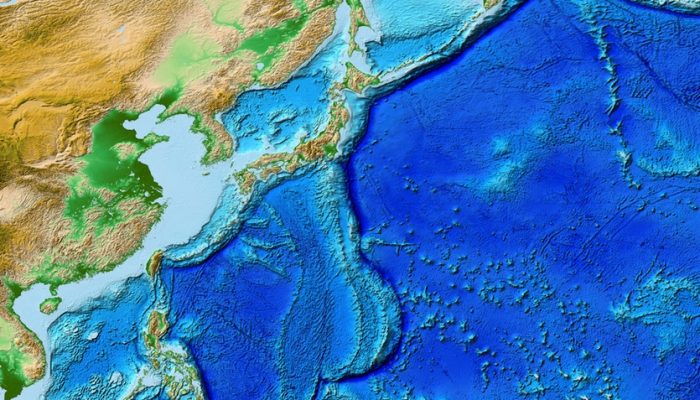