Are you an Early Career Scientist (ECS) working on the topics of the Geochemistry, Mineralogy, Petrology, and Volcanology (GMPV) division? Do you enjoy engaging with scientific communities and communicating with a broad audience? The GMPV division of the EGU is looking for a new Editor-in-Chief to lead our blog team! On the GMPV Blog, we share scientific insights from the GMPV fields and curiositi ...[Read More]
La Soufrière de Guadeloupe: Past Eruptions and Ongoing Activity
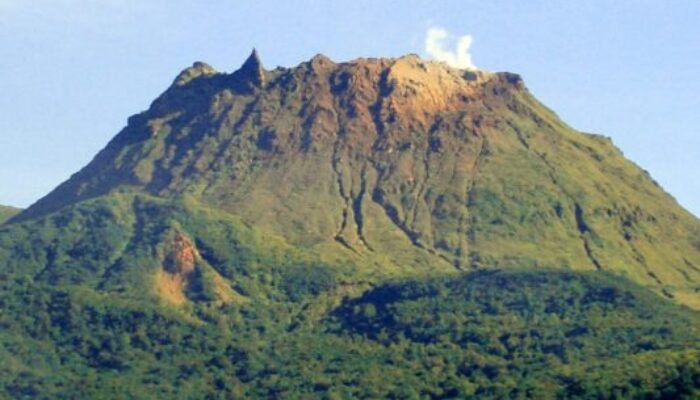
La Soufrière de Guadeloupe is an andesitic stratovolcano located in the southern part of Basse-Terre, within the Guadeloupe archipelago (Eastern Caribbean). It towers over the towns of Sainte-Claude and Basse-Terre. Nearly 50 years ago, this region faced severe consequences from a phreatomagmatic eruption, forcing the evacuation of 80,000 people. Since then, the volcano has been regularly monitore ...[Read More]
GMPV ECS Campfires – Meet the GMPV GA24 awardees! September 12th @11 am CET
The first edition of the Geochemistry, Mineralogy, Petrology and Volcanology Campfires of the 2024/2025 season is right at the door and will take place on Thursday September 12th at 11 am CET on Zoom. This edition will be a special Scientific Campfire, during which the three GMPV ECS awardees of the 2024 General Assembly will present their latest work to the community. Our speakers for this editi ...[Read More]
THE CHALLENGES OF DATING – GEOLOGISTS’ VERSION (part one)
When it comes to dating profiles, minerals can put up a really good face at first. But, as some of the users in dating apps out there, they might not be worthy of a second try, or maybe not even of a first depending on what your intentions are… To help out, we will tackle some of the main minerals used in geochronology, as if they were featured on online dating apps, plus some ‘red flags’ from pre ...[Read More]