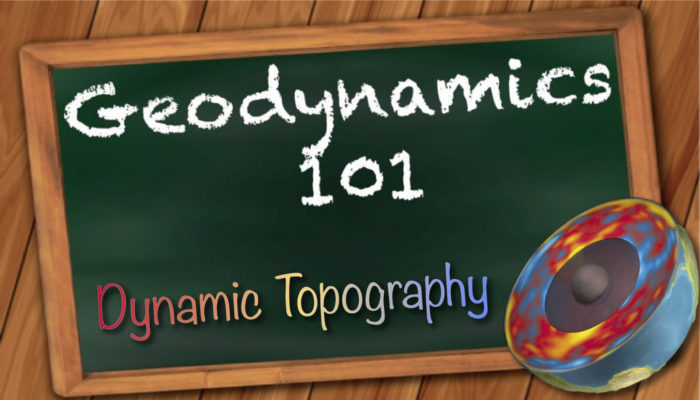
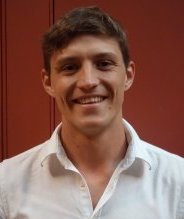
Research fellow Fred Richards, Imperial College London, UK.
The Geodynamics 101 series serves to showcase the diversity of research topics and/or methods in the geodynamics community. In this week’s post, Fred Richards explains how ‘Dynamic Topography’ is used in the Geosciences, and discusses the knowns, unknowns, and the challenges ahead.
Since shortly after its tumultuous formation 4.5 billion years ago, Earth has been steadily cooling, with its interior separating into a dense iron-rich core and a silicate mantle. Within the mantle, heat is continuously transported to the surface via convective upwellings, this hot material then conductively cools and densifies, before being returned to the depths in the form of slabs (Figure 1). This internal flow pattern has long been linked to horizontal plate motions and the supercontinent cycle; however, careful analysis of the rock record has begun to reveal evidence for transient vertical motions as well. There is now increasing recognition that this “dynamic” topography can cause geologically rapid changes in elevation (~100s of metres per million years) [1]. This “bounciness” means that dynamic topography can have major impacts on surface processes such as landscape evolution, ocean circulation, palaeoshoreline formation and ice sheet stability [2-5].
Although it is now generally accepted that mantle flow plays an important role in generating surface topography, there has been some (occasionally quite fierce!) debate about the strength of the dynamic topography signal at different lengthscales, and how fast it evolves. A portion of the disagreement comes down to how dynamic topography should be defined. Some researchers include lithospheric isostasy in their definition (i.e., topography generated by thickness and density variations in the mechanically rigid lithosphere) [6], while, at the other end of the spectrum, some exclude all isostatically compensated topography, including the component supported by density anomalies in the convecting mantle [7] (Figure 2). None of these definitions is necessarily incorrect, what’s important is to use a consistent definition when comparing model outputs and observations. The other main source of debate concerns the large discrepancies between observational estimates of dynamic topography and model predictions [8,9]. At the largest lengthscales (~104km), many model-based predictions produce more than double the amount of dynamic topography inferred from observations, and the reasons for this mismatch remain unclear. However, disagreement at shorter lengthscales (~103km) has largely been resolved by accounting for density anomalies in the shallow upper mantle (<300 km) and recognising that ~25% of the observed signal is from lithospheric isostasy, rather than buoyancy anomalies in the convecting part of the mantle [10,11]. This improvement in our ability to accurately model these shallower, smaller-scale density anomalies is important, as they cause the most rapid changes in dynamic topography and therefore have the greatest impact on palaeoclimatic and geomorphologic studies.
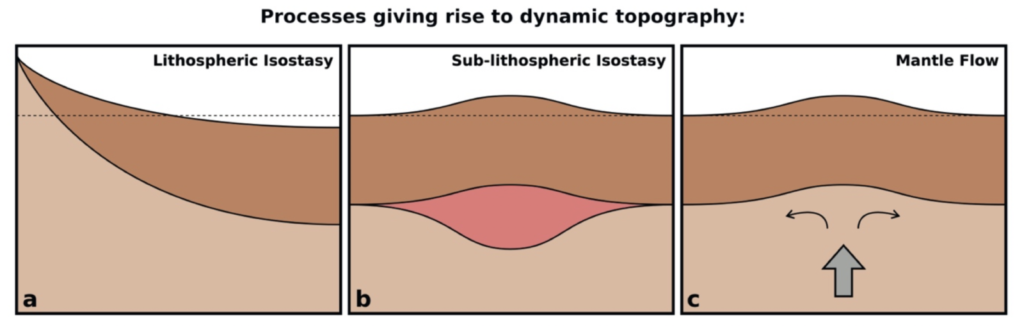
Figure 2: Processes that generate dynamic topography. a) Lithospheric isostasy. b) Sub-lithospheric isostasy. c) Mantle flow. Depending on their adopted definition of dynamic topography, different researchers may include all three (e.g., Forte et al. [12]), exclude a) (e.g., Davies et al. [10]), or exclude both a) and b) (e.g., Molnar et al. [7]). From Hoggard et al. [13].
Known unknowns?
There are many! However, I’ve picked out what I think are the three key ones. The first is that we still don’t know what proportion of present-day continental topography is dynamically supported because there is so much uncertainty on the isostatic contribution. This is because, over Earth history, the continental lithosphere has been modified by magmatism and mangled by tectonics. The best techniques we currently have for measuring continental crustal thickness generally have ± 2 km error, while our knowledge of internal chemical variability is very limited, relying mainly on uncertain conversions between seismic wave speeds and density. As a result, uncertainties in the amplitude of continental dynamic topography currently dwarf the likely amplitude of the signal itself. We therefore need more accurate constraints on continental density structure. Fortunately, new “thermochemical tomography” techniques that integrate mantle xenolith geochemistry and geophysical observables are starting to show significant promise in this regard [14].
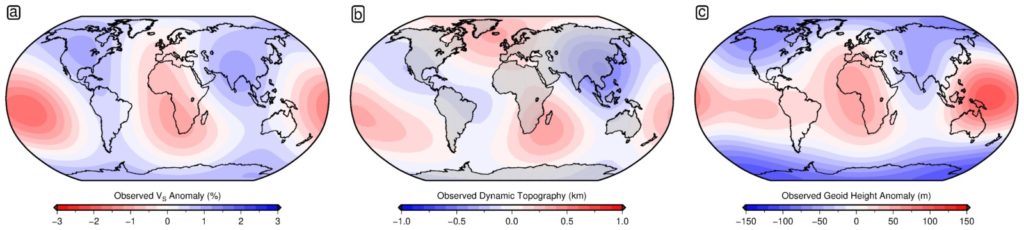
Figure 3: Long-wavelength correlations between deep mantle shear wave velocities and geodynamic observables. a) Vs anomalies from S40RTS (spherical harmonic degrees 1-3 [15]). b) Same for observational estimates of dynamic topography [8]. c) Same for observed non-hydrostatic geoid [16].
The second known unknown is that we currently struggle to simultaneously fit the Earth’s ± 100 m geoid undulations and ± 700 m long-wavelength (~10,000 km) dynamic topography with mantle convection models. Strong spatial correlation between geoid and dynamic topography highs and the location of LLVPs – continent-sized regions of slow seismic wave speed at the base of the mantle – suggests that a better understanding of their density structure may help to resolve this issue as, although dynamic topography is weakly sensitive to deep mantle structure, the ratio of geoid to dynamic topography provides a relatively strong constraint (Figure 3). In particular, if LLVPs are not purely thermal features, but instead host chemical heterogeneities, it may be possible to find LLVP buoyancy structures that can match geodynamic observations. Our current understanding of LLVP density is limited by seismic tomographic resolution, the relative insensitivity of seismic wave speeds to chemical versus thermal anomalies, and the different sensitivities of the observations we can use to map their internal structure. Nevertheless, recent joint inversions of seismic and geodynamic data, using a range of mantle viscosity models, suggest that the general thermochemical characteristics of LLVPs can be determined [17]. Inclusion of additional constraints will likely help to improve the picture further.
A third significant challenge is that our knowledge of how dynamic topography has changed through time remains patchy. From an observational standpoint, a wide range of constraints can be used to investigate uplift and subsidence, including stratigraphic architecture at continental margins, preserved sea-level indicators, thermochronologic records of erosion, and the shapes of river profiles [18–20]. However, it can be difficult in some cases to isolate vertical motions due to dynamic topography from those related to glacial isostatic adjustment, eustatic sea-level change, active tectonics, sedimentation and plate flexure. From a geodynamic modelling perspective, the sparsity of these constraints currently limits their usefulness for validating model predictions. Instead, time-dependent convection models are generally constrained by plate motion histories and the final state of the mantle, i.e., its tomographically inferred present-day buoyancy structure. Recent advances in this direction, such as adjoint-based inversions of mantle flow history, have improved our ability to “retrodict” mantle convection over ~10-million-year timescales, but these solutions are non-unique and dependent on assumed mantle rheology, which remains highly uncertain [21,22]. Even so, rates of dynamic topography change are quite sensitive to mantle viscosity, suggesting that, if we can improve our observational constraint on convective vertical motions, we may be able to narrow in on a much smaller subset of possible mantle convection histories.
Frontiers
While significant challenges remain, exciting new avenues for research have been opened up. One example is the study of ice age palaeoclimate, which has tended to assume – with the exception of glacial isostatic adjustment and localised tectonic activity – that Earth’s surface has remained a passive, non-deforming substrate [23]. This common assumption is undermined by the existence of rapidly evolving dynamic topography and we may need to revise our present understanding of certain climatic periods, especially those that occurred millions of years before present. The mid-Pliocene warm period (MPWP; ~ 3 million years ago) is of particular interest as it represents the last time in Earth history that atmospheric CO2concentrations were similar to the present. Estimates of sea-level during this time are therefore used to tune ice sheet models and sea-level forecasts. However, uncertainty on these estimates is currently large due to the wide range of MPWP palaeoshoreline elevations (~15–65 m) and this spread is likely caused, at least in part, by changes in dynamic topography [24] (Figure 4). In certain locations where agreement between model predictions and observations is good, it may be possible to correct palaeoshoreline elevations for dynamic topography to get more accurate constraints on global mean sea-level during this critical period.
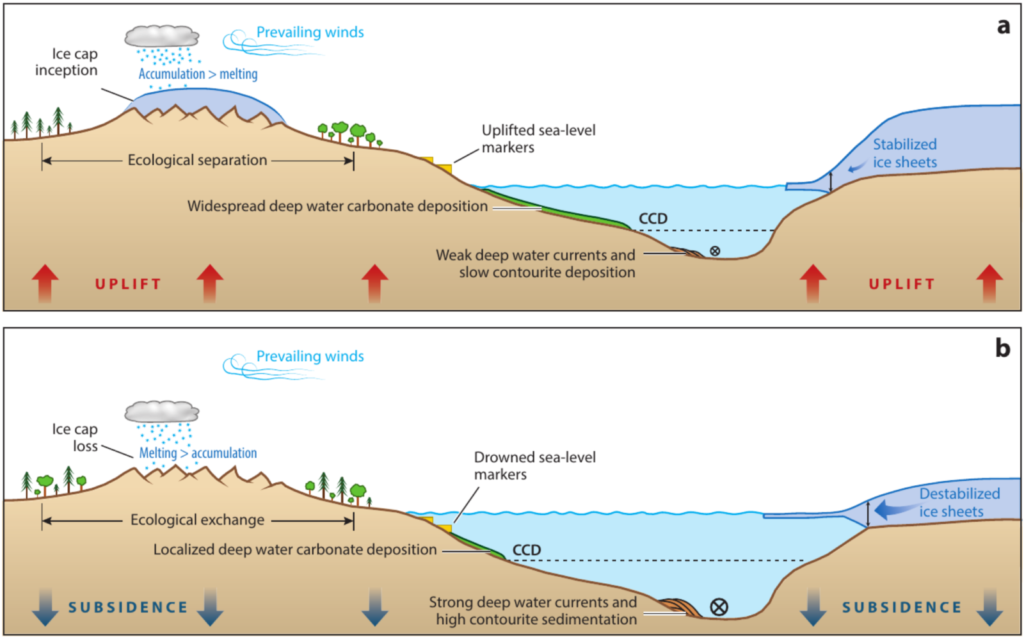
Figure 4: Potential impacts of dynamic topography on geological observations used to constrain palaeoclimate. a) Lithosphere is uplifting through time. b) Lithosphere subsides over time. From Mitrovica et al. [25].
In addition to its effect on mid-Pliocene sea-level records, the impact of topography changes on the intrinsic stability of continental ice sheets during this time is an important area of research. Decreases in bedrock elevation can result in an inland retreat of ice-sheet grounding lines (i.e., where the ice sheet loses contact with basement rocks and begins to float), reducing the ice storage capacity of the continents, and it has been suggested that this may be partly responsible for higher sea-levels during the MPWP [5]. By contrast, uplift can help to stabilise ice sheets and may even help to trigger their formation by modifying atmospheric and oceanic circulation patterns (Figure 4). For example, the inception of Northern Hemisphere glaciation at the end of the MPWP has been linked to uplift of the North Atlantic region and associated changes in ocean gateway elevations and climatic sensitivity to solar insolation forcing [26–28].
Clearly, developing a full understanding of the impact of dynamic topography on surface process will require a lot more work, and large uncertainties on the internal viscosity and density structure of the mantle remain. However, expansion of palaeotopographic data compilations and integration of these constraints with sophisticated inverse modelling techniques will allow us to steadily refine our knowledge of past mantle states and rheology [29]. It will not be long before we can start to give more definitive answers to the palaeoclimate questions outlined above and many more.
References [1] Hartley, R. A., Roberts, G. G., White, N., & Richardson, C., 2011. Transient convective uplift of an ancient buried landscape., Nature Geoscience, 4, 562–565. [2] Braun, J., Robert, X., & Simon-Labric, T., 2013. Eroding dynamic topography, Geophysical Research Letters, 40(8), 1494–1499. [3] Parnell-Turner, R. E., White, N. J., Henstock, T. J., Murton, B. J., Mclennan, J., & Jones, S. M., 2014. A continuous 55-million-year record of transient mantle plume activity beneath Iceland, Nature Geoscience, 7(12), 914–919. [4] Rovere, A., Raymo, M. E., Mitrovica, J. X., Hearty, P. J., O’Leary, M. J., & Inglis, J. D., 2014. The Mid-Pliocene sea-level conundrum: Glacial isostasy, eustasy and dynamic topography, Earth and Planetary Science Letters, 387, 27–33. [5] Austermann, J., Pollard, D., Mitrovica, J. X., Moucha, R., Forte, A. M., DeConto, R. M., Rowley, D. B., & Raymo, M. E., 2015. The impact of dynamic topography change on Antarctic ice sheet stability during the mid-Pliocene warm period, Geology, 43(10), 927–930. [6] Glišović, P., & Forte, A. M., 2016. A new back-and-forth iterative method for time-reversed convection modeling: Implications for the Cenozoic evolution of 3-D structure and dynamics of the mantle, Journal of Geophysical Research: Solid Earth, 121(6), 4067–4084. [7] Molnar, P., England, P. C., & Jones, C. H., 2015. Mantle dynamics, isostasy, and the support of high terrain, Journal of Geophysical Research: Solid Earth,120(3), 1932–1957. [8] Hoggard, M. J., White, N. J., & Al-Attar, D., 2016. Global dynamic topography observations reveal limited influence of large-scale mantle flow, Nature Geoscience, 9(6), 456–463. [9] Yang, T., & Gurnis, M., 2016. Dynamic topography, gravity and the role of lateral viscosity variations from inversion of global mantle flow, Geophysical Journal International, 207(2), 1186–1202. [10] Davies, D. R., Valentine, A. P., Kramer, S. C., Rawlinson, N., Hoggard, M. J., Eakin, C. M., & Wilson, C. R., 2019. Earth’s multi-scale topographic response to global mantle flow, Nature Geoscience, 12(10), 845-850. [11] Richards, F. D., Hoggard, M. J., White, N. J., & Ghelichkhan, S., 2020. Quantifying the relationship between short-wavelength dynamic topography and thermomechanical structure of the upper mantle using calibrated parameterization of anelasticity,Journal of Geophysical Research: Solid Earth, 125(9), e2019JB019062 [12] Forte, A. M., Peltier, W. R., Dziewonski, A. M., & Woodward, R. L., 1993. Dynamic surface topography: A new interpretation based upon mantle flow models derived from seismic tomography, Geophysical Research Letters, 20(3), 225–228. [13] Hoggard, M.J., J. Austermann, C. Randel, & S. Stephenson, in press. Observational estimates of dynamic topography through space and time, in Mantle Convection and Surface Expressions (Eds: H. Marquardt, M. Ballmer, S. Cottaar, & J. Konter), AGU Geophysical Monograph Series, Washington, DC. [14] Afonso, J. C., Rawlinson, N., Yang, Y., Schutt, D. L., Jones, A. G., Fullea, J.,, & Griffin, W. L., 2016. 3-D multiobservable probabilistic inversion for the compositional and thermal structure of the lithosphere and upper mantle: III. Thermochemical tomography in the Western-Central U.S., Journal of Geophysical Research: Solid Earth, 121(10), 7337–7370. [15] Ritsema, J., Deuss, A. A., Van Heijst, H. J., & Woodhouse, J. H., 2011. S40RTS: a degree-40 shear-velocity model for the mantle from new Rayleigh wave dispersion, teleseismic traveltime and normal-mode splitting function measurements. Geophysical Journal International, 184(3), 1223-1236. [16] Chambat, F., Ricard, Y., & Valette, B., 2010. Flattening of the Earth: further from hydrostaticity than previously estimated. Geophysical Journal International, 183(2), 727–732. [17] Lu, C., Forte, A. M., Simmons, N. A., Grand, S. P., Kajan, M. N., Lai, H., & Garnero, E. J., 2020. The sensitivity of joint inversions of seismic and geodynamic data to mantle viscosity. Geochemistry, Geophysics, Geosystems, 21(4), e2019GC008648. [18] Czarnota, K., Hoggard, M. J., White, N. J., & Winterbourne, J., 2013. Spatial and temporal patterns of Cenozoic dynamic topography around Australia, Geochemistry, Geophysics, Geosystems, 14(3), 634–658. [19] Rowley, D. B., Forte, A. M., Moucha, R., Mitrovica, J. X., Simmons, N. A., & Grand, S. P., 2013. Dynamic topography change of the eastern United States since 3 million years ago, Science, 340(6140), 1560–1564. [20] Fernandes, V. M., Roberts, G. G., White, N., & Whittaker, A. C., 2019. Continental scale landscape evolution: A history of North American topography, Journal of Geophysical Research: Earth Surface. 124(6), 2689–2722. [21] Ghelichkhan, S., & Bunge, H.-P., 2016. The compressible adjoint equations in geodynamics: Derivation and numerical assessment, International Journal on Geomathematics,7, 1–30. [22] Li, D., Gurnis, M., & Stadler, G., 2017. Towards adjoint-based inversion of time dependen tmantle convection with nonlinear viscosity, Geophysical Journal International,209(1), 86–105. [23] Miller, K. G., Kominz, M. A., Browning, J. V., Wright, J. D., Mountain, G. S., Katz, M. E., Sugarman, P. J., Cramer, B. S., Christie-Blick, N., & Pekar, S. F., 2005. The Phanerozoic record of global sea-level change, Science, 310(5752), 1293–1298. [24] Raymo, M.E., Mitrovica, J.X., O’Leary, M.J., DeConto, R. M., & Hearty, P.J., 2011. Searching for eustasy in Pliocene sea-level records, Nature Geoscience,4(3), 28–32. [25] Mitrovica, J. X., Austermann, J., Coulson, S. L., Creveling, J. R., Hoggard, M. J., Jarvis, G.T., & Richards, F. D.,2020. Dynamic Topography and Ice Age Paleoclimate, Annual Reviews of Earth and Planetary Sciences, 48(1), 585–62. [26] Parnell-Turner, R. E., White, N. J., McCave, N., Henstock, T. J., Murton, B. J., & Jones, S. M., 2015. Architecture of North Atlantic contourite drifts modified by transient circulation of the Icelandic mantle plume, Geochemistry, Geophysics, Geosystems, 16(10), 3414–3435. [27] Daradich, A., Huybers, P., Mitrovica, J. X., Chan, N.-H., Austermann, J., 2017. The influence of true polar wander on climate and glacial inception in North America, Earth and Planetary Science Letters, 461, 96–104. [28] Coulson., S., Austermann J., Hoggard, M. J., Richards, F. D., Mitrovica, J. X., 2018. The role of dynamic topography on glacial inception in North America. Presented at AGU Fall Meeting,Dec. 10–14, Washington, DC, DI51B-0005. [29] Fernandes, V. M., & Roberts, G. G., 2020, August 16. Fernandes and Roberts (2020) - preprint. https://doi.org/10.31223/osf.io/vgf5e.
He Tang
I like it. It is clear. After read it, I begin to understand the concept of dynamic-topography. Thank you!