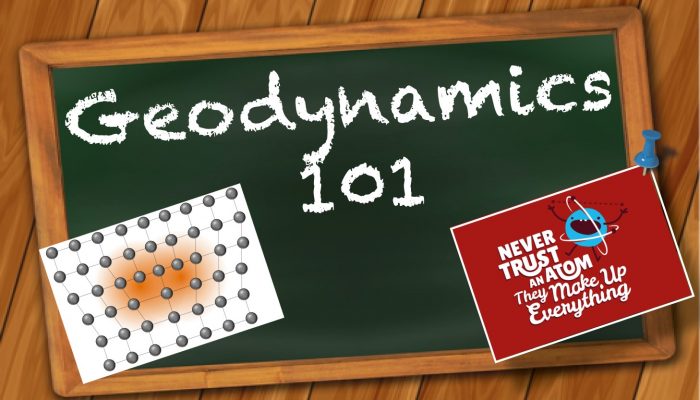
The Geodynamics 101 series serves to showcase the diversity of research topics and methods in the geodynamics community in an understandable manner. We welcome all researchers – PhD students to Professors – to introduce their area of expertise in a lighthearted, entertaining manner and touch upon some of the outstanding questions and problems related to their fields. For our first ‘101’ for 2018, we have an entry by postdoctoral researcher Elvira Mulyukova from Yale University about rheology and deformation occurring on atomic scales … it’s a fun and informative read indeed! Do you want to talk about your research? Contact us!
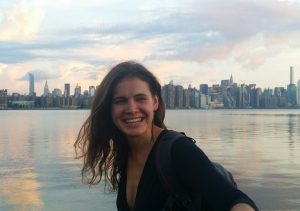
Elvira Mulyukova, Yale University
Most of us have an intuitive understanding that different materials resist being moved, or deformed, to different degrees. Splashing around in the mud is more energy-consuming (and fun, but never mind that) than in the water, and splashing around in the block of concrete is energy-intensive bordering on deadly. What are the physical reasons for these differences?
For Earth materials (rocks), the answer lies in the restless nature of their atoms: the little buggers constantly try to sneak out of their crystal lattice sites and relocate. Some are more successful at it than others, making those materials more easily deformable. A lattice site is really just other atoms surrounding the one that is trying to escape. You see, atoms are like a bunch of introverts: each is trying to escape from its neighbours, but doesn’t want to get near them. The ones that do escape have to overcome a temporary discomfort (or an increase in their potential energy, for those physically inclined) of getting close to their neighbours. This requires energy. The closer the neighbours – the more energy it takes to get past them. When you exert force on a material, you force some of the neighbours to be further away from our potential atomic fugitive, making it more likely for the atom to sneak in the direction of those neighbours. The fun part (well, fun for nerds like me) is that it doesn’t happen to just one atom, but to a whole bunch of them, wherever the stress field induced by the applied force is felt. A bunch of atoms escaping in some preferential direction is what we observe as material deformation. The more energy you need to supply to induce the mass migration of atoms – the stronger the material. But it’s really a question of how much energy the atom has to begin with, and how much energy is overall needed to barge through its detested neighbuors. For example, when you crank up the temperature, atoms wiggle more energetically and don’t need as much energy supplied from external forcing in order to escape – thus the material gets weaker.
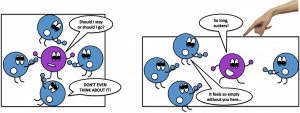
“A lattice site is really just other atoms surrounding the one that is trying to escape. You see, atoms are like a bunch of introverts: each is trying to escape from its neighbours, but doesn’t want to get near them.” cartoon by Elvira Mulyukova
One more thing. Where are the atoms escaping to? Well, there happen to be sanctuaries within the crystal lattice – namely, crystalline defects such as vacancies (aka point defects, where an atom is missing from the otherwise ordered lattice), dislocations (where a whole row of atoms is missing), grain boundaries (where one crystal lattice borders another, which is tilted relative to it) and other crystalline imperfections. These regions are sanctuaries because the lattice is more disordered there, which allows for larger distances in-between the neighbors. When occupying a regular lattice site – the atom is sort of trapped by the crystalline order. Think of the lattice as an oppressive regime, and the crystalline defects as liberal countries that are welcoming refugees. I don’t know, is this not the place for political metaphors? *…whistling and looking away…*
Ok, enough anthropomorphisms, let’s get to the physics. If this is the last sentence you’ll read in this blog entry, let it be this: rocks are made up of atoms that are arranged into crystal lattices (i.e., ordered rows and columns of atoms), which are further organized into crystal grains (adjacent crystals tilted relative to each other); applying force to a material encourages atoms to move in a preferential direction of the largest atomic spacing, as determined by the direction of the applied force; the ability of the lattice sites to keep their atoms in place (call it a potential energy barrier) determines how easily a material deforms. Ok, so it was more like three sentences, but now you know why we need to get to the atomic intricacies of the matter to understand materials macroscopic behaviour.
Alright, so we’re applying a force (or stress, which is simply force per area) to a material and watch it deform (a zen-inducing activity in its own right). We say that a material behaves like a fluid when its response to the applied stress (and not just any stress, but differential stress) is to acquire a strain rate (i.e., to progressively shorten or elongate in one direction or the other at some rate). On geological time scales, rocks behave like fluids, and their continuous deformation (mass migration of atoms within a crystal lattice) under stress is called creep.
The resistance to deformation is termed viscosity (let’s denote it µ), which basically tells you how much strain rate (˙e) you get for a given applied differential stress (τ). Buckle up, here comes the math. For a given dimension (say x, and for the record – I’ll only be dealing with one dimension here to keep the math symbols simple, but bear in mind that µ, ˙e and τ are all tensors, so you’d normally either have a separate set of equations for each dimension, or some cleverly indexed symbols in a single set of equations), you have:
So if I’m holding a chunk of peridotite with a viscosity of 1020 Pa s (that’s units of Pascal-seconds, and that’s a typical upper mantle viscosity) and squeezing it in horizontal direction with a stress of 108 Pa (typical tectonic stress), it’ll shorten at a rate of 5 · 10-13 s-1 (typical tectonic rates). A lower viscosity would give me a higher strain rate, or, equivalently, with a lower viscosity I could obtain the same strain rate by applying a smaller stress. If at this point you’re not thinking “oh, cool, so what determines the viscosity then?,” I failed massively at motivating the subject of this blog entry. So I’m just gonna go ahead and assume that you are thinking that. Right, so what controls the viscosity? We already mentioned temperature (let’s call it T), and this one is a beast of an effect. Viscosity depends on temperature exponentially, which is another way of saying that viscosity depends on temperature hellavulot. To throw more math at you, here is what this dependence looks like:
where R = 8.3144598 J/K/mol (that’s Joule per Kelvin per mol) is the gas constant and E is the activation energy. Activation energy is the amount of energy that an atom needs to have in order to even start thinking about escaping from its lattice site, which of course depends on the potential energy barrier set up by its neighbours. Let’s say your activation energy is E = 530·103 J mol-1 . If I raised your temperature from 900 to 1000 K (that’s Kelvin, and those are typical mid-lithospheric temperatures), your viscosity would drop by a factor of ∼ 1000. That’s a three orders of magnitude drop.
Like I said, helluvalot. If instead you had a lower activation energy, say E = 300 · 103 J mol-1 , the same temperature experiment would bring your viscosity down by a factor of ∼ 50, which is less dramatic, but still significant. It’s like running through peanut butter versus running through chocolate syrup (running through peanut butter is a little harder… I clearly need to work on my intuition-enhancing examples). Notice, however, that while the temperature dependence is stronger for materials with higher activation energies, it is more energy-consuming to get the creep going in those materials in the first place, since atoms have to overcome higher energy barriers. There’s more to the story. Viscosity also depends on pressure (call it P), which has a say in both the energy barrier the atoms have to overcome in order to escape their neighbours, as well as how many lattice defects (called sanctuaries earlier) the atoms have available to escape to. The higher the pressure, the higher the energy barrier and the fewer lattice sanctuaries to resort to, thus the higher the viscosity. Throwing in the pressure effect, viscosity goes as:
The exact dependence of viscosity on pressure is determined by V – the activation volume.
Alright, we’re finally getting to my favourite part – the atoms’ choice of sanctuary sites. If the atomic mass migration happens mainly via point defects, i.e., by atoms hopping from one single lattice vacancy to another, the deformation regime is called diffusion creep. As atoms hop away, vacancies accumulate in regions of compressive stress, and fewer vacancies remain in regions of tensional stress. Such redistribution of vacancies can come about by atoms migrating through the bulk of a crystal (i.e., the interior of a grain, which is really just a crystal that is tilted relative to its surrounding crystals), or atoms migrating along the boundary of a crystal (i.e., a grain boundary). In both cases, the rate at which atoms and vacancies get redistributed depends on grain size (let’s denote it r). The larger the grains – the more distance an atom has to cover to get from the part of the grain that is being compressed to the part that is under tension. More math is due. Here is what the viscosity of a material deforming by diffusion creep looks like:
Exponent m depends on whether the atoms are barging through the bulk of a grain (m = 2), or along the grain boundaries (m = 3). What’s that new symbol B in the denominator, you ask? That’s creep compliance (in this case – diffusion creep compliance), and you two have already met, sort of. Creep compliance specifies how a given creep mechanism depends on pressure and temperature:
For diffusion creep of upper mantle rocks, I typically use m = 3, B0 ∼ 13 µmm MPa-1 s-1 (which is just a material-specific prefactor), Ediff = 300 · 103 J mol-1 and Vdiff = 5 cm3 mol-1 from Karato and Wu (1993). Sometimes I go bananas and set Vdiff = 0 cm3 mol-1 , blatantly ignoring pressure dependence of viscosity, which is ok as long as I’m looking at relatively modest depth-ranges, like a few tens of kilometers.
At sufficiently high stresses, a whole row of atoms can become mobilized and move through the crystal, instead of the meagre one-by-one atomic hopping between the vacancies. This mode of deformation is called dislocation creep. Dislocations are really just a larger scale glitch in the structure of atoms (compared to vacancies). They are linear lattice defects, where a whole row of atoms can be out of order, displaced or missing. It requires more energy to displace a dislocation, because you are displacing more than one atom, but once it’s on the move, it accommodates strain much more efficiently than in the each-atom-for-itself diffusion creep regime. As the material creeps, dislocations get born (nucleated), get displaced and get dead (annihilated). Dislocations don’t care about grain size. What they do care about is stress. Stress determines the rate at which dislocations appear, move and disappear. I know you saw it coming, more math, here is the dislocation creep viscosity:
Exponent n dictates the stress dependence of viscosity. Stress dependence of dislocation creep viscosity is a real pain, making the whole thing nonlinear and difficult to use in a geodynamical model. Not impossible, but rage-inducingly difficult. Say you’re trying to increase the strain rate by some amount, so you increase the stress, but then the viscosity drops, and suddenly you have a monster of a strain rate you never asked for. Ok, maybe it’s not quite this bad, but it’s not as good as if the viscosity just stayed constant. You wouldn’t be able to have strain localization, form tectonic plate boundaries and develop life on Earth then, but maaaan would you be cracking geodynamic problems like they were peanuts! I’m derailing. Just like all the other creeps, dislocation creep has its own compliance, A, that governs its dependence on pressure and temperature:
For dislocation creep of upper mantle rocks, I typically use n = 3, A0 = 1.1 · 105 MPa-n s -1 (which is just a material-specific prefactor), Edisl = 530 · 103 J mol -1 and Vdiff = 20 cm3 mol-1 , similar to Karato and Wu (1993). Just like for diffusion creep, I sometimes just set Vdisl = 0 cm3 mol-1 to keep things simple.
We’re almost done. Allow me one last remark. A rock has an insane amount of atoms, crystal grains and defects, all subject to local and far-field conditions (stress, temperature, pressure, deformation history, etc). A typical rock is therefore heterogeneous on the atomic (nano), granular (micro) and outcrop (meter) scales. Thus, within one and the same rock, deformation will likely be accommodated by more than just one mechanism. With that in mind, and sticking to just two deformation mechanisms described above, we can mix it all together to get:
This is known as composite rheology, where we assumed that the strain rates accommodated by diffusion and dislocation creep can be simply summed up, like so:
Alright. If you got down to here, I salute you! Next time you’re squeezing a peridotite, or splashing in the mud, or running through peanut butter – give a shout out to those little atoms that enable you to do such madness. And if you want to get to the physics of it all, you can find some good introductory texts in Karato (2008); Turcotte and Schubert (2002).
References S. Karato. Deformation of earth materials: an introduction to the rheology of solid earth. Cambridge Univ Pr, 2008. S. Karato and P. Wu. Rheology of the upper mantle: a synthesis. Science, 260(5109):771–778, 1993. D.L. Turcotte and G. Schubert. Geodynamics. Cambridge Univ Pr, 2002.