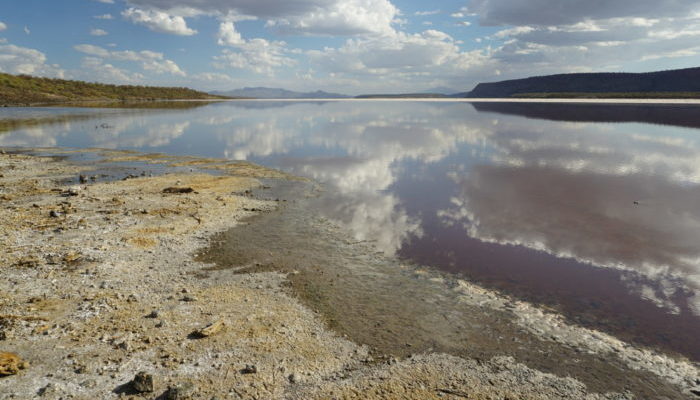
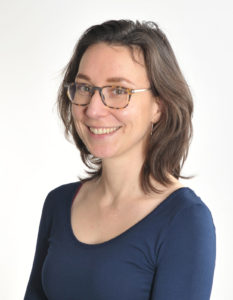
Dr. Anne Glerum from GFZ Potsdam.
This week in News & Views, Anne Glerum, postdoc at GFZ Potsdam, discusses how her numerical models support a lithosphere-driven mechanism for the rotation of large continental microplates, like Victoria in the East African Rift System.
The East African Rift System (EARS) is a newly forming divergent boundary between the Nubian and Somalian plates (Fig. 1). The plate boundary system includes several continental rift arms and one or more smaller microplates. According to GPS data (e.g. Saria et al. 2014), while Somalia and the other microplates rotate clockwise relative to Nubia, the Victoria microplate is rotating counter-clockwise.
Previous hypotheses suggested that this distinctive rotation is driven by the interaction of a mantle plume with the microplate’s cratonic keel and the rift system (Calais et al. 2006, Koptev et al. 2015, 2016). While plumes can help localize deformation at crustal and lithospheric heterogeneities (e.g. Beniest et al. 2017), with the help of 3D time-dependent upper-scale numerical models, we propose that the configuration of weaker and stronger lithospheric regions predominantly controls the rotation of continental microplates and that of Victoria in particular.
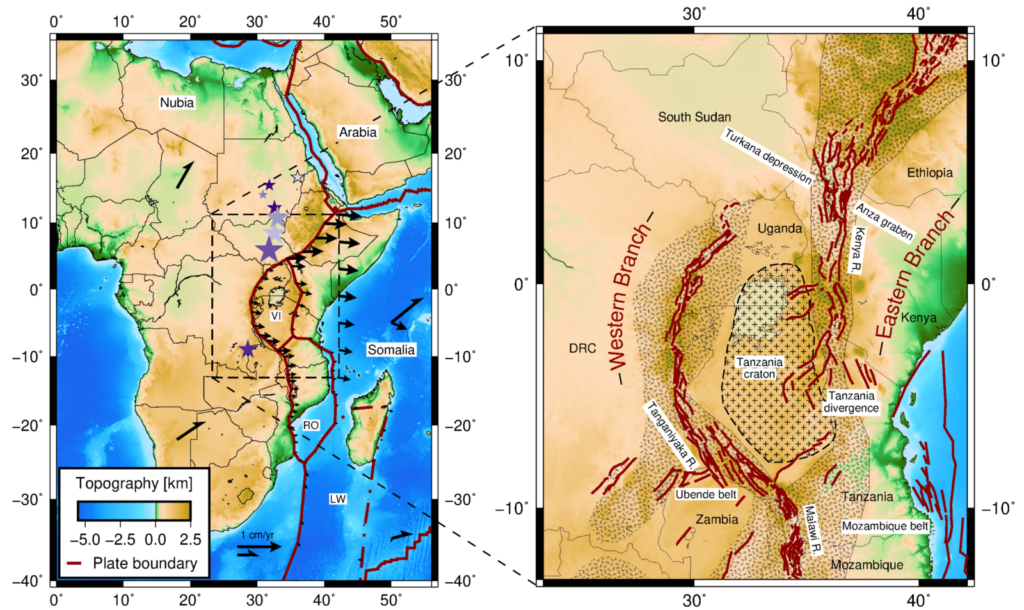
Figure 1. The East African Rift System (EARS). Left: General overview of the plate boundary system showing the Victoria (VI), Rovuma (RO) and Lwandle (LW) microplates. Black full-head vectors show relative velocities, while vectors with a halved head indicate absolute plate motions in a global moving hotspot reference frame (Kreemer et al. 2014). Right: Zoom-in of the region of interest and numerical model domain showing the individual faults of the EARS in red (GEM Active fault database), the rheologically weak mobile belts (dotted areas) and the Tanzania craton (plusses in dashed outline). Modified from Glerum et al. (2020).
In the EARS, Proterozoic mobile belts, like the Mozambique belt (Fig. 1), guide the localization and propagation of the rift arms. The particular configuration of the mechanically weaker mobile belts led to the curved, partly overlapping Eastern and Western rift branches of the rift system. At the same time, rheologically stronger regions are formed by for example the failed Anza rift and the Tanzania craton, at which the Eastern rift branch diverges. This particular configuration of lithospheric strength under the ~E-W extensional motion of the major tectonic plates, transmitted to the microplate along the stronger areas, induces Victoria’s rotation.
We demonstrated this edge-driven mechanism (Schouten et al. 1993) for large continental microplates with generic numerical models on the scale of the EARS (2100x2700x300 km). We then tested what particular geometry of partly overlapping mobile belts leads to the fastest rotation – an ellipsoidal geometry of EARS proportions. With a mobile belt configuration modelled after the EARS (Fig. 2), we then investigated the additional effect of stronger than average lithosphere on the rotation as well as that of a gradual decrease in prescribed extension velocity towards the south, as seen in the EARS (black arrows in Fig. 1a). The combination of EARS belt configuration, stronger craton and failed rift regions, and a gradual velocity decrease (Fig. 2f) predicts a rotation pole of the model Victoria microplate closest to the recent rotation pole of Saria et al. (2014) based on GPS data as well as earthquake slip directions and other geological indicators (Fig. 2g).
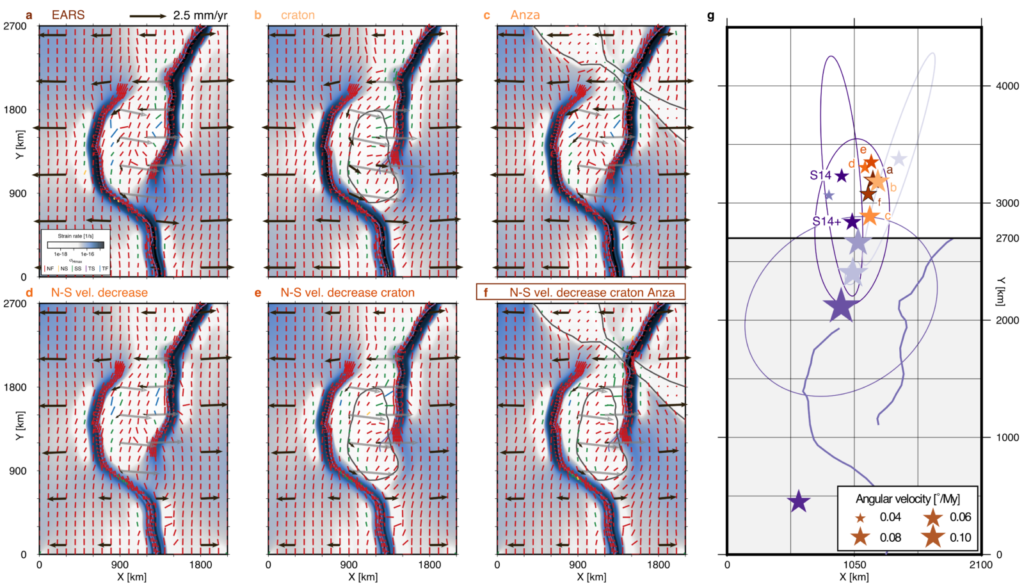
Figure 2. a-f) Top view of numerical model predictions of velocity, strain rate and the direction of maximum horizontal compressive stress coloured according to faulting regime (red: NF normal faulting, yellow: NS normal and strike–slip faulting, green: SS strike–slip faulting, purple: TS thrust and strike–slip faulting, blue: TF thrust faulting). g) Predicted Victoria microplate rotation poles (brown stars) versus rotation poles obtained from block modelling of GNSS data (purple stars). S14: Saria et al. (2014) GPS velocity based pole. S14+: Saria et al. (2014) pole based on GPS velocities, earthquake slip vectors and geological indicators. Modified from Glerum et al. (2020).
The good match between predicted and observed Victoria rotation poles brought us to compare our stress predictions to the World Stress Map (Heidbach et al. 2016; Fig. 3a&c). Both show predominantly normal faulting, with the maximum horizontal compressive stress direction changing in orientation along the curved rift branches. Notably, the oblique mobile belts (with respect to the overall extension direction) and the induced rotation lead to stress orientations that locally deviate from the N-S directions expected from the prescribed E-W regional extension. Only in the most oblique section of the Western Branch, transient phases of strike-slip faulting are predicted.
The model evolution allows us to synthesise several kinematic interpretations of the EARS (e.g. Morley 2010, Chorowicz 2006, Delvaux et al. 2012) as follows: In the overall E–W extending system of partly overlapping arcuate rift branches that follow lithospheric weak zones, drag of the major plates along stronger sides of the microplate intrinsically leads to local WNW–ESE extension directions in the rift segments. In the oblique sections, normal faulting thus occurs at small angles to the trend of the rift and the pre-existing weaknesses, with some strike-slip motion. Nevertheless, motion is predominantly accommodated by normal faulting. Small-scale sources such as basement fabric can locally deflect the stress field (Morley 2010) such that even the nonoverlapping, oblique Tanganyika-Rukwa-Malawi segment deforms under a mostly normal-faulting regime.
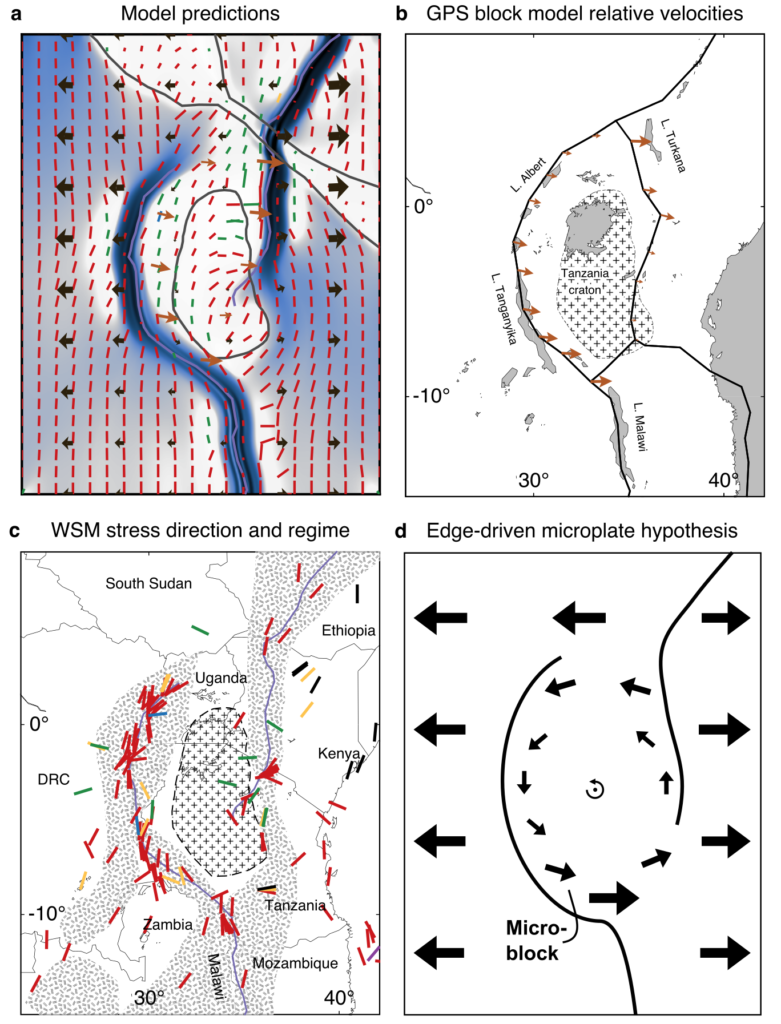
Figure 3. Hypothesis, model predictions and observations. a) Model predictions (see Fig. 2f) with brown vectors showing the relative velocity with respect to the plate to the west. b) Relative velocity predictions w.r.t. the plate to the west based on the Euler pole obtained from block modelling by Saria et al. (2014). c) Maximum horizontal stress directions coloured according to tectonic regime (see legend in Fig. 2a) from the World Stress Map (Heidbach et al. 2016). d) The edge-driven mechanism for continental microplate rotation. Modified from Glerum et al. (2020).
These insights provide exciting opportunities for. As the heterogeneity of the lithosphere plays such a large role in the deformation of East Africa, we are currently working on incorporating observational data directly into our model setup, such that we can zoom in on second-order dynamic processes affecting crust and lithosphere tectonics. At the same time, we are using the lithosphere-scale edge-driven mechanism to interpret the rotations of some of the many more continental microplates on Earth.
References Beniest, A. and others (2017). Two-Branch Break-up Systems by a Single Mantle Plume: Insights from Numerical Modeling. Geophys. Res. Lett., 44(19), 9589–9597. Calais, E. and others (2006). Kinematics of the East African Rift from GPS and earthquake slip vector data. Geological Society, London, Special Publications, 259(1), 9–22. Chorowicz, J. (2005). The East African rift system. J. African Earth Sci., 43(1–3), 379–410. Delvaux, D. and others (2012). Geodynamic significance of the TRM segment in the East African Rift (W-Tanzania): Active tectonics and paleostress in the Ufipa plateau and Rukwa basin. J. Struct. Geol., 37, 161–180. Glerum, A., Brune, S., Stamps, D. S., & Strecker, M. R. (2020). Victoria continental microplate dynamics controlled by the lithospheric strength distribution of the East African Rift. Nat. Commun., 11(1), 2881. Heidbach, O. and others (2016). World Stress Map Database Release. GFZ Data Services. Koptev, A. and others (2015). Dual continental rift systems generated by plume-lithosphere interaction. Nat. Geosci., 8(5), 388–392. Koptev, A. and others (2016). Contrasted continental rifting via plume-craton interaction: Applications to Central East African Rift. Geosci. Front., 7(2), 221–236. Kreemer, C. and others (2014). A geodetic plate motion and Global Strain Rate Model. Geochemistry, Geophys. Geosystems, 15(10), 3849–3889. Morley, C. K. (2010). Stress re-orientation along zones of weak fabrics in rifts: An explanation for pure extension in “oblique” rift segments? Earth Planet. Sci. Lett., 297(3–4), 667–673. Saria, E. and others (2014). Present-day kinematics of the East African Rift. J. Geophys. Res. Solid Earth, 119(4), 3584–3600. Schouten, H. and others (1993). Edge-driven microplate kinematics. J. Geophys. Res., 98(B4), 6689–6701.