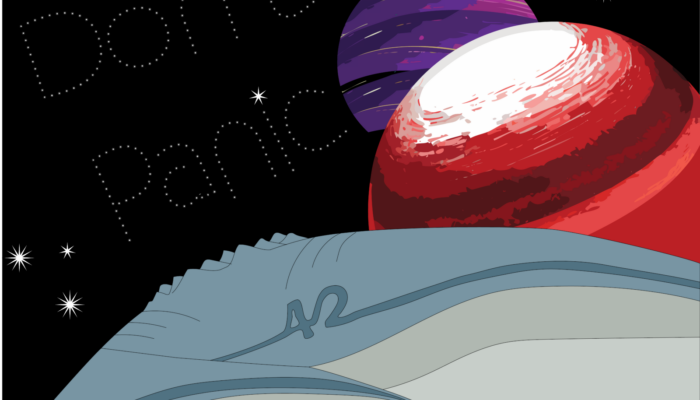
“The Hitchhiker’s Guide to the Galaxy” had the answer; we think we have the right question. This week, Ajay Kumar from IISER Pune, India, will take us on a journey to the depths of the Earth’s lithosphere – a world as mysterious as the farthest reaches of the Universe. We will see what the thickness of the Earth’s crust can tell us about the balance between the internal energy of lithospheric plates and the tectonic forces acting at their boundaries, how that balance might shape the evolution of Earth-like planets, and why 42 might be the answer.
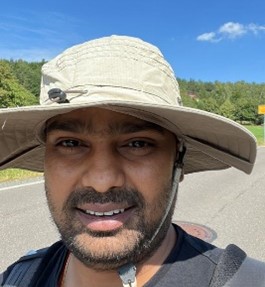
Ajay Kumar is a geophysicist who is interested in thermo-chemical and thermomechanical evolution of the lithosphere. He recently moved from Section 4.5 Subsurface Process Modelling GFZ Potsdam to IISER Pune, India, where he is working as an Assistant Professor in the Department of Earth and Climate Science. His contact and more information could be found at https://sites.google.com/site/kumarajay6763/home.
What is common between the “The Hitchhiker’s Guide to the Galaxy” and the solid Earth? The answer is “42”. While the “The Hitchhiker’s Guide to the Galaxy” leaves readers/audience to contemplate what the answer means, in this blog, based on our recent work (Kumar et al., 2023), we give the meaning to this in the context of solid Earth dynamics.
Continental lithosphere, crust plus the lithospheric mantle, covers ~30% of the Earth’s surface above sea level. Broadly, continental areas show geologically two kinds of regions: regions that are stable (e.g., cratons), and regions that are tectonically active (e.g., orogens, mountains). Orogenic regions represent a phase of the Wilson Cycle where two continental tectonic plates interact, leading to spatially wide deformation of the continental lithosphere and the interaction zone can no longer be considered as rigid. This is exemplified by the Alpine Himalayan Collision Zone (AHCZ), which starts from Gibraltar Strait in the west to the Indo-Burman subduction zone in the east (Figure 1a).
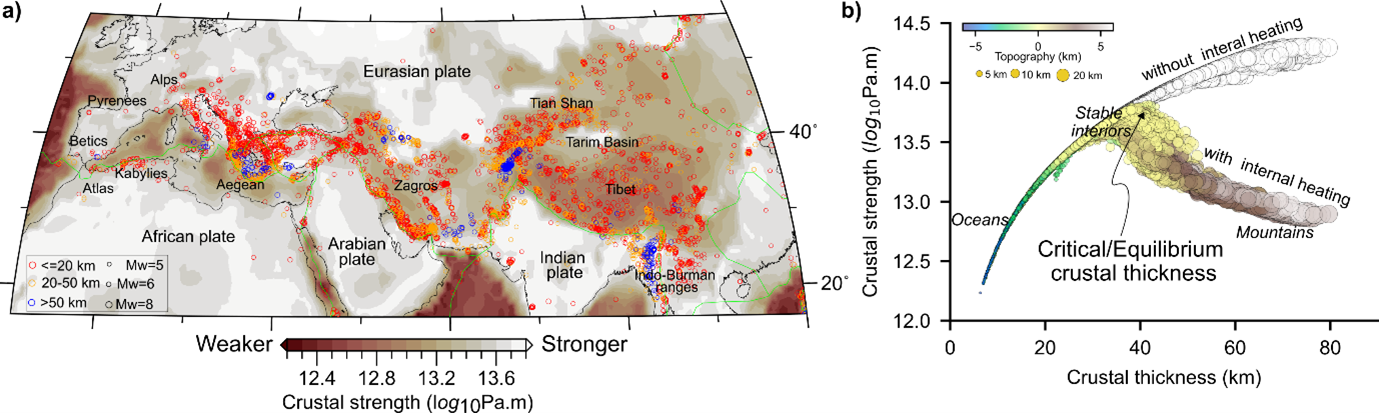
Figure 1. a) Map of the Alpine Himalayan Collision Zone (AHCZ) showing the long-term strength of the crust together with seismicity from ISC- GEM catalogue (Storchak et al., 2013), colour coded by depth and scaled in size using magnitude. Kinematic plate boundaries from Bird (2003) are plotted by green curves. In the continental regions, warmer colours indicate thicker and weaker crust corresponding to the mountains/orogens. Lighter colours indicate a stronger crust of the stable continental interiors. b) Plot of crustal strength vs crustal thickness (Crust 1.0, Laske et al., 2013) highlighting positive correlation below ~42 km and negative above. Coloured circles show the corresponding topography for the individual data points and are scaled in size for upper-crustal thickness. White circles indicate a scenario where radiogenic heat production in the crust is not considered and demonstrates that crustal strength would continuously increase with thickness if the internal heating were switched off. Figure modified after Kumar et al. (2023).
Continental deformation is a complex interplay of tectonic and gravitational forces (e.g., Houseman & Houseman, 2010; P. Rey, 2001; P. F. Rey & Houseman, 2006), which is a central phenomenon to the evolution of Earth’s lithosphere. This interaction changes depending on the thickness and strength of the lithosphere, which is controlled primarily by temperature. Hence deviations from a thermal equilibrium and the re-establishment of the latter (thermal relaxation) are of key importance.
We looked at the present-day data-derived thermomechanical state of the AHCZ and found that a stable thermodynamic equilibrium exists between the internal energy of the Earth’s lithospheric plates and the tectonic plate boundary forces. Such an equilibrium manifests itself through a critical thickness of the continental crust of ~42 km, which corresponds approximately to the global average of today’s continental crust on Earth and is also typical of stable old cratons (Figure 1b). Deviations from this critical thickness lead to continental deformation, in particular along those parts of the AHCZ where the continental crust is severely thickened, leading to a diffuse distribution of deformation and related seismicity. The key factor here is radiogenic heat, i.e., the heat produced within the crust when radioactive elements decay. If the continental crust is thicker than ~42 km, the additional radiogenic heat causes the internal energy to increase, making the thickened regions weaker, and be dissipated in the form of diffuse deformation, for example in Tibet where crustal thickness reaches ~80 km (Figure 2b). These differences in thickness explain why seismicity across the AHCZ collision zone is over a wider area, which is in contrast to the localised deformations occurring along the plate boundaries.
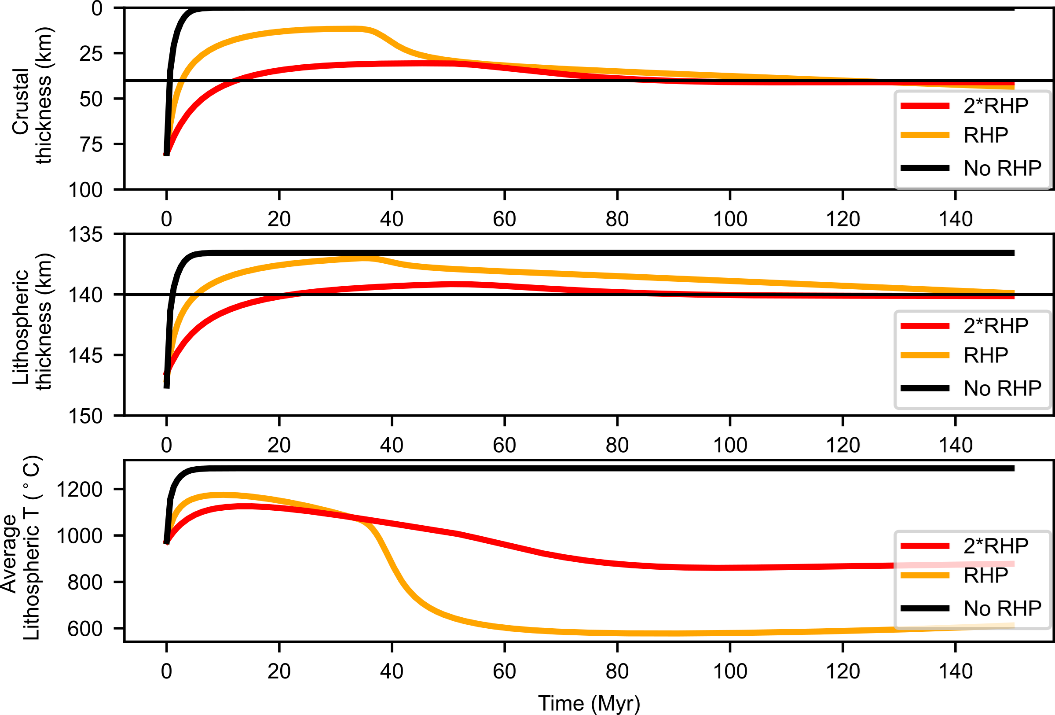
Figure 2. Evolution of an orogenic lithospheric 1D column with time. Top panel shows the time evolution of crustal thickness, middle panel shows the evolution of lithospheric thickness and bottom panel shows the evolution of depth average lithospheric temperature. Curves with different colour show experiments with varying amount of radiogenic heat production (RHP). Black curves correspond to the experiment where RHP is not considered. Note the very early evolution to 0 crustal thickness. Orange curves show the experiment with representative value of RHP in the continental crust and red curves that with double the representative of RHP.
It is known that orogens would evolve to lose their high topography or produce new oceanic basins completing the Wilson Cycle (e.g., Dewey, 1988; Wolf et al., 2022), but what are the key controlling factors and related timescales? To understand this, we considered the critical crustal thickness to be a state of equilibrium and considered the variation in the crustal thicknesses as perturbation to it. The time evolution of such perturbations is driven by the temperature-dependent potential energy with respect to the equilibrium. The analogy that we draw here is that of a damped harmonic oscillator. A feedback loop exists between the thermal relaxation (cooling/heating) and mechanical relaxation (strain) because of the temperature dependence of potential energy (i.e., driving energy, through thermal expansion) and temperature dependence of strain rate (e.g., thermally activated viscous creep). We found that the radiogenic heat production plays a crucial role in the evolution in that it helps orogenic lithosphere to evolve back to the equilibrium crustal thickness within an order of 100 million years, rather than leading to runaway extension (formation of oceanic basin) when radiogenic heat production is not considered (Figure 2). Heat from radiogenic decay decreases the driving potential energy: e.g., decreasing the crustal thinning rates and allowing the thermal cooling to take over. These results allowed us to draw a genetic link between the thermo-chemical state of the Earth’s crust and the evolution of silicate Earth-like planets (Figure 3).
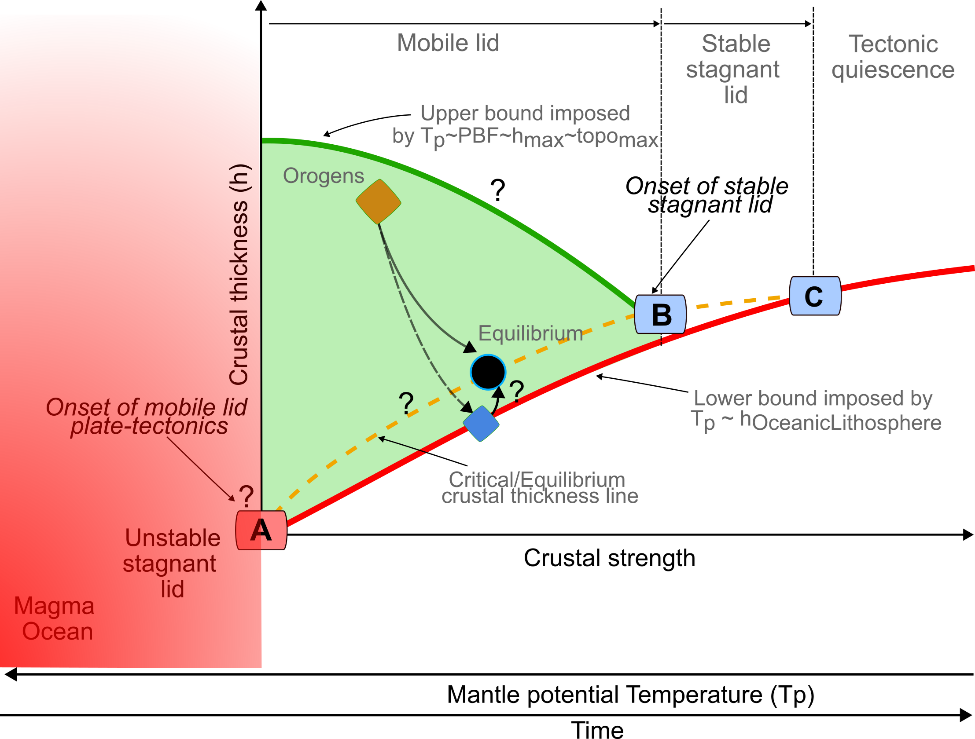
Figure 3. Schematic diagram depicting the evolution of an Earth-like planet in terms of its crustal thickness (h), crustal strength, and mantle potential temperature (Tp). The light green area bounded by red line and green line represents the possible parameter space in terms of crustal thickness that may evolve in response to plate boundary forces (PBF) and prevailing Tp. Any perturbation to the stable equilibrium state (black circle), such as thickening during orogenesis (orange diamond) or thinning (blue diamond), causes the system to evolve around this stable equilibrium state, the path of which is a function of the crustal rheology, composition, and internal energy content. The solid black arrows show a typical evolutionary path from orogens to oceanic lithosphere, whereas the dashed arrow shows a system that goes towards runaway extension (rifting) in case of external perturbations (e.g., plumes from deeper mantle). Modified after Kumar et al. (2023).
Studies have highlighted a correlation between the onset of plate tectonics and the degree of crustal differentiation on Earth (e.g., Dhuime et al., 2015). The evolution of plate tectonics is intricately linked to the thermochemical state of the continental plate, as reflected in the critical crustal thickness (Figure 3). The mantle potential temperature (Tp) serves as a crucial indicator of the energy driving plate tectonics. Changes in Tp influence lithospheric thickness, density contrast, and coupling across the lithosphere-asthenosphere boundary. (1) a phase of very high internal energy that is too hot for plates to form, (2) a phase of mobile lid plate-tectonics during which the forces in response to the internal energy and the plate boundary forces are balanced and (3) a phase of a stagnant lid when the internal energy is smaller than the plate boundary forces and the increased strength of the lithospheric plates prevents plate deformation (Figure 3). Thus, the critical crustal thickness is influenced by Tp, highlighting its significance in governing plate tectonics on Earth-like planets.
The answer 42 km suggests a dynamic equilibrium between the plate strength and driving forces for present-day Earth. Would this number be representative of other silicate planets (e.g., Venus or Mars) or would it have different value and why? The schematic evolutionary phase-space in Figure 3 suggests that this equilibrium should evolve with mantle potential temperature, with implications for the continental crust growth, gradual versus rapid (Korenaga, 2018). I do not know the answer to these questions yet (maybe someone does and would love to know), but I am hitchhiking with “The Hitchhiker’s Guide to the Galaxy”.
References: [1] Bird, P. (2003). An updated digital model of plate boundaries. Geochemistry, Geophysics, Geosystems, 4(3). https://doi.org/10.1029/2001GC000252 [2] Dewey, J. F. (1988). Extensional Collapse of Orogens. Tectonics, 7(6), 1123–1139. https://doi.org/10.1029/TC007i006p01123 [3] Dhuime, B., Wuestefeld, A., & Hawkesworth, C. J. (2015). Emergence of modern continental crust about 3 billion years ago. Nature Geoscience, 8(7), 552–555. https://doi.org/10.1038/ngeo2466 [4] Houseman, G. A., & Houseman, D. K. (2010). Stability and periodicity in the thermal and mechanical evolution of the early continental lithosphere. Lithos, 120(1–2), 42–54. https://doi.org/10.1016/j.lithos.2010.09.001 [5] Korenaga, J. (2018). Crustal evolution and mantle dynamics through Earth history. Philosophical Transactions of the Royal Society A: Mathematical, Physical and Engineering Sciences, 376(2132). https://doi.org/10.1098/rsta.2017.0408 [6] Kumar, A., Cacace, M., & Scheck-Wenderoth, M. (2023). Thermodynamics of continental deformation. Scientific Reports, 13(1), 19920. https://doi.org/10.1038/s41598-023-47054-3 [7] Laske, G., Masters, G., Ma, Z., & Pasyanos, M. (2013). Update on CRUST1.0---A 1-degree global model of Earth’s crust. EGU General Assembly 2013, 15, 2658. http://meetingorganizer.copernicus.org/EGU2013/EGU2013-2658.pdf [8] Rey, P. (2001). From lithospheric thickening and divergent collapse to active continental rifting. Geological Society, London, Special Publications, 184(1), 77–88. https://doi.org/10.1144/GSL.SP.2001.184.01.05 [9] Rey, P. F., & Houseman, G. (2006). Lithospheric scale gravitational flow: the impact of body forces on orogenic processes from Archaean to Phanerozoic. Geological Society, London, Special Publications, 253(1), 153–167. https://doi.org/10.1144/GSL.SP.2006.253.01.08 [10] Storchak, D. A., Giacomo, D. Di, Bondár, I., Engdahl, E. R., Harris, J., Lee, W. H. K., Villaseñor, A., & Bormann, P. (2013). Public release of the ISC-GEM global instrumental earthquake catalogue (1900-2009). Seismological Research Letters, 84(5), 810–815. https://doi.org/10.1785/0220130034 [11] Wolf, S. G., Huismans, R. S., Braun, J., & Yuan, X. (2022). Topography of mountain belts controlled by rheology and surface processes. Nature, 606(7914), 516–521. https://doi.org/10.1038/s41586-022-04700-6