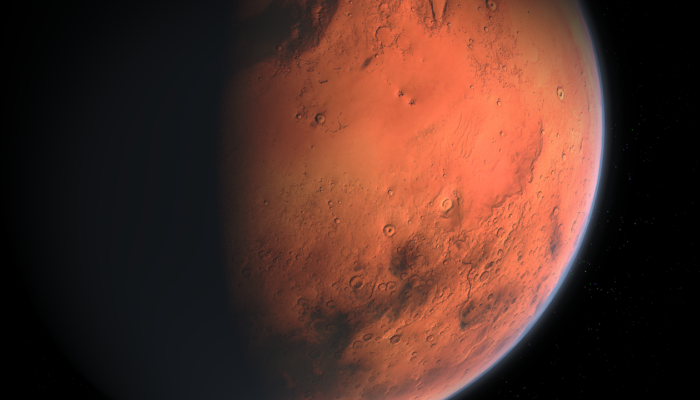
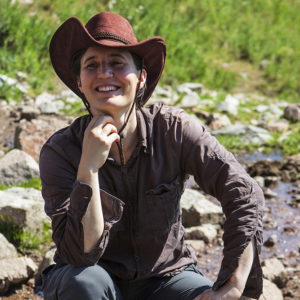
Giulia Magnarini, Post Doctoral Researcher at the Natural History Museum, London
Landslides can be impressively huge and fast and can occur on all sorts of places including asteroids, rocky moons and Mars! Giulia Magnarini Post Doctoral Researcher at the Natural History Museum in London writes all about these gigantic landslides and the clues they could hold into the martian past!
Gigantic landslides are ubiquitous in our solar system. Indeed, Earth, Mars, Mercury, Venus, asteroid Vesta, dwarf planet Ceres, rocky moons such as the Moon and Phobos and the icy moons of the outer Solar System such as Callisto, Iapetus and Charon, are all known hosts to landslides that are up to tens of kilometres long and hundreds of metres thick. These impressive features, called long runout landslides are characterised by their large volumes (> 106 m3, that is 400 Olympic size swimming pools!) and the ability to move away from the head scarp at velocities that can exceed 100 km/h for up to tens of km, along sub-horizontal surfaces. The mobility of landslides is commonly expressed as a ratio of their height drop (H) and their horizontal extent (L). Long runout landslides are characterised by an H/L ratio significantly lower than 0.6. The lower the H/L ratio the more mobile these landslides are. While it is well-known that the presence of water enhances the mobility of landslides in general, curiously long runout landslides such as the El Magnifico and Saidmarreh landslides (Figure 1) show no evidence of water involvement in their emplacement. Indeed, the origin of these huge, hypermobile landslides across the solar system is one of the most intriguing open questions in the field!
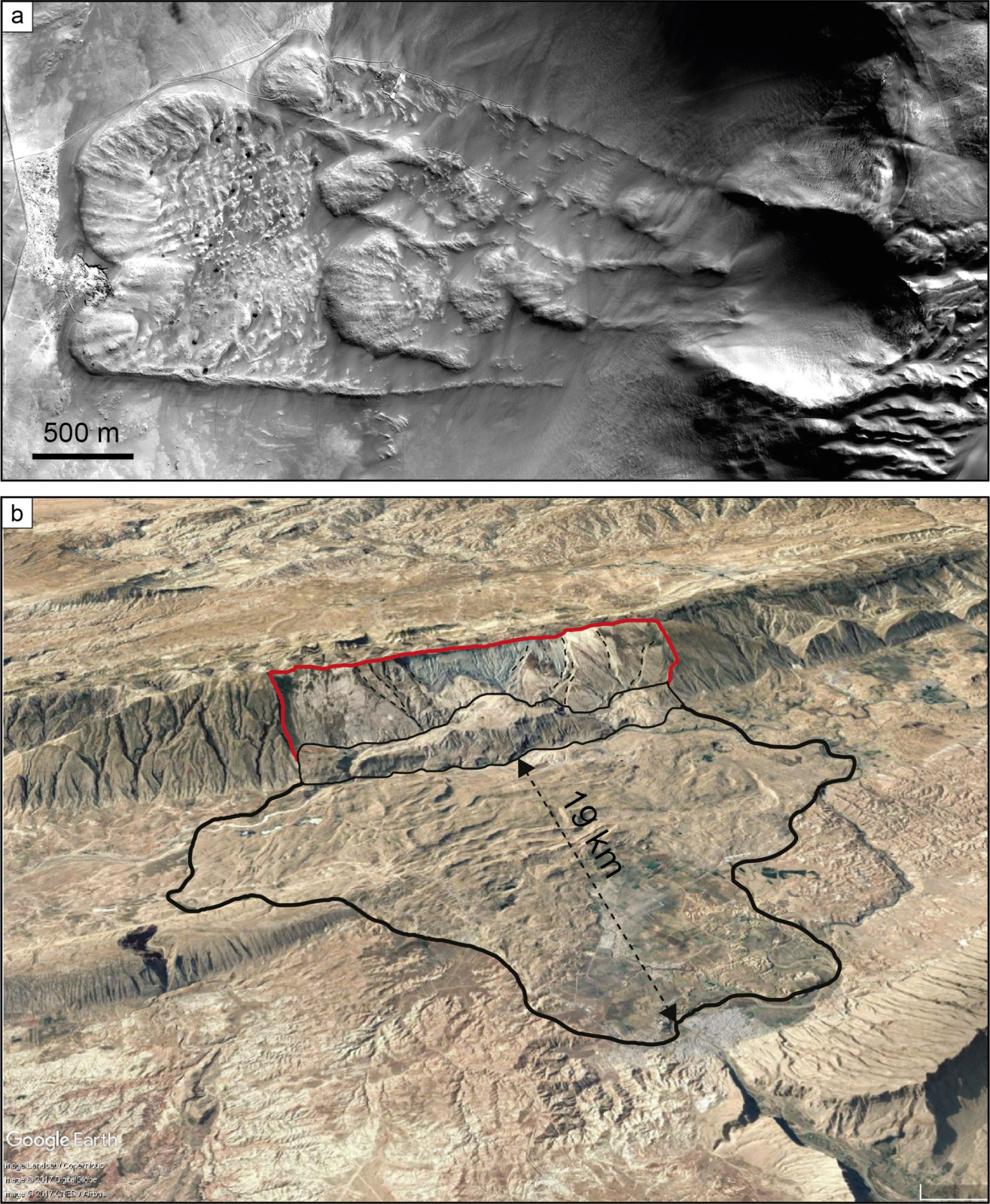
Figure 1: Two examples of terrestrial long runout landslides that show no evidence of water involvement in their emplacement. A) The El Magnifico landslide, in Northern Atacama, Chile (orthoimage created from stereo-images acquired by Pleiades satellites, ©CNES, 2013, distribution Airbus Defence and Space). B) The Saidmarreh landslides, in the Zagros Mountains, Iran.
Long runout landslides are one of the most characteristic landforms visible on the surface of Mars (Figure 2). The first images of landslides on Mars came from the NASA Mariner 9 mission in 1971. However, it was the Viking missions programme in the mid- and late 70’s that really revealed the extraordinary morphologies of the martian landslides and the role these play in shaping the ~4000 km-long canyon Valles Marineris (Lucchitta, 1979). The Valles Marineris located few degrees south of the martian equator hosts hundreds of gigantic landslides: some of which are entirely blocking the hundred-km wide valleys; while others do so only partially; and others form a stack of overlapping deposits (Figure 2). Despite a wide variety of form, the long runout landslides that characterise the chasmata (i.e., the deep, elongated, and steep-sided depressions) of the Valles Marineris feature longitudinal ridges. These are linear structures that extend in the direction of the landslide emplacement for almost the entire length of the deposit (Figure 2). On Earth, longitudinal ridges are commonly observed in long runout landslides emplaced on glaciers (for example, the Sherman Glacier landslide) and their morphological similarity with martian ridges has led to the hypothesis that ice had been extensively present in the Valles Marineris! Since the age of the long runout landslides in Valles Marineris appears to span throughout the majority of martian history (from as early as 3.5 Gya to as recently as 50 Mya, according to crater counting method (Quantin et al., 2004)), this theory therefore, implies a consistent and stable martian ice cover at these latitudes for most of the planet’s history. However, given the equatorial location of Valles Marineris and our current knowledge of the martian climatic history, this seems highly unlikely.
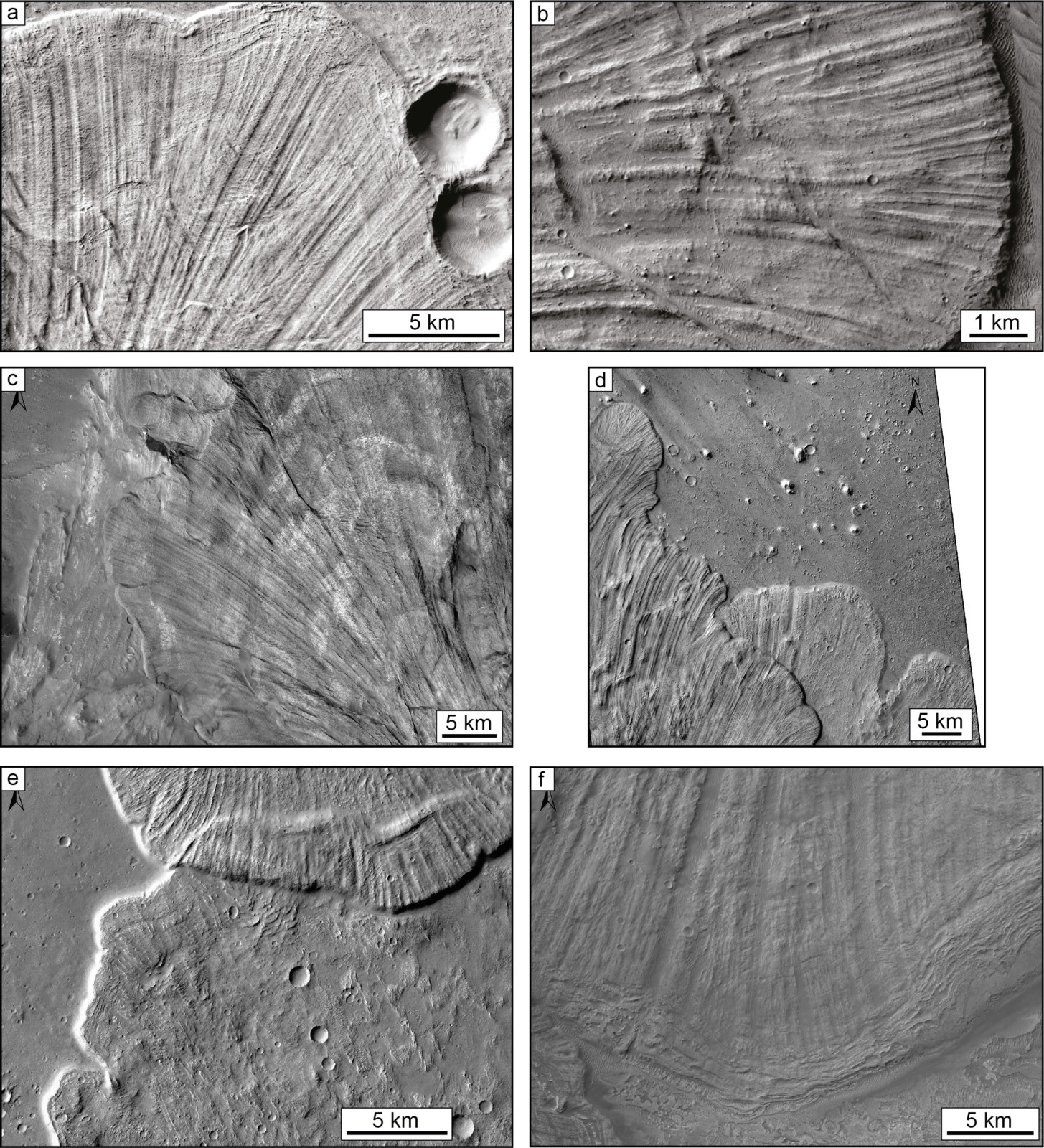
Figure 2: Examples of the remarkably well-preserved longitudinal ridges in martian long runout landslides. They extend for the entire length of the landslide deposit. C, d, and e show examples of overlapping landslides in Ganges Chasma: the younger landslides run up an older landslide deposit; the longitudinal ridges that characterise the younger deposits remain unperturbed despite the landslide superimposing another deposit. (Image credit: NASA/JPL/MSSS).
Interesting Longitudinal ridges are also found in terrestrial volcanic landslides (e.g., Valderrama, 2016) and in long runout landslides that were not emplaced on ice, for example, the El Magnifico landslide in Northern Atacama on Earth and the Tsiolkovskiy crater landslide on the Moon. As a matter of fact, morphometric studies (that is the measurement of morphological parameters such as height, thickness etc.) of longitudinal ridges in these terrestrial and lunar landslides (Magnarini et al., 2021a; 2021b), and a martian landslide in Valles Marineris (Magnarini et al., 2019) have found a scaling relationship between the wavelength of the ridges and the thickness of the landslide deposit that is in agreement with the results obtained in ice-free laboratory experiments in rapid granular flows (Forterre and Pouliquen, 2001). These studies show that an icy bottom surface is not necessary for the development of longitudinal ridges in long runout landslides; and that the mechanism for the formation of longitudinal ridges is environment- and scale-independent. This means that when we see landslides with longitudinal ridges we should not jump straight away to the conclusion that ice was involved!
Although Valles Marineris could arguably be the best place on Mars where to find the most spectacular examples of long runout landslides, it is not the only region where they can be found. The latest catalogue counts thousands of martian long runout landslides between 60° N and 60° S. According to a recent study (Crosta et al., 2018), landslides increase mobility with latitude. The reason behind this could be attributed to the latitudinal distribution of ice. Alternatively, other work suggests that clay-bearing material forming taluses and valley floor sediments could have also facilitated the runout of landslides in Valles Marineris (Watkins et al., 2015; 2020. Note, Jessica Watkins is now a NASA astronaut!). Some landslides in Valles Marineris may have also been subaqueous. Interestingly, changes in the morphology of landslide deposits that occur at a consistent elevation within mid-latitude martian craters have been attributed to the past presence of a stationary water level fed by a regional groundwater system (Salese et al., 2019). This variety of locations at which long runout landslides have occurred on Mars may suggest that different conditions could play a part in enhancing their mobility.
This becomes even more obvious if we consider the wide variety of environments across the solar system that record the presence of long runout landslides. Closer to home, terrestrial long runout landslides are found in disparate environments, from subaerial continental to volcanic, submarine, glacial, involving different lithologies. The only thing that they seem to have in common is their exceptional runout caused by a dramatic reduction of friction that takes place during these catastrophic events. A list of mechanisms that have been proposed to explain the hypermobility of long runout landslides: in addition to ice (Dufresne and Davies, 2009; De Blasio, 2011), other low-friction surfaces are suggested, such as evaporites (De Blasio, 2011) and frictional melt (e.g., Erismann et al., 1977; Legros et al., 2000). Overpressured fluids at the base of the moving slides have also been suggested as a possible friction reduction mechanism, resulting in a hovercraft effect (e.g., Vardoulakis, 2000; Goren et al., 2010); similarly vibrations could also be able to fluidise the entire sliding mass (Melosh, 1979).
Many advances in our understanding of these enigmatic and fascinating features have been made in the past twenty years or so. Contributions have come from areas of research such as the physics of granular flows, computational modelling, fault mechanics, and planetary sciences. Studying long runout landslides on other planetary bodies has the advantage that their geomorphological record is well-preserved, because of the reduced erosion rates. If you then have high resolution imagery available, this becomes a powerful asset. Thanks to the NASA Mars Reconnaissance Orbiter mission, we have now a 6 m/px global and repetitive coverage of the martian surface, and this allows the production of 20 m/px digital elevation models to be used for detailed morphometric analysis of long runout landslides and their morphological features (Figure 3).
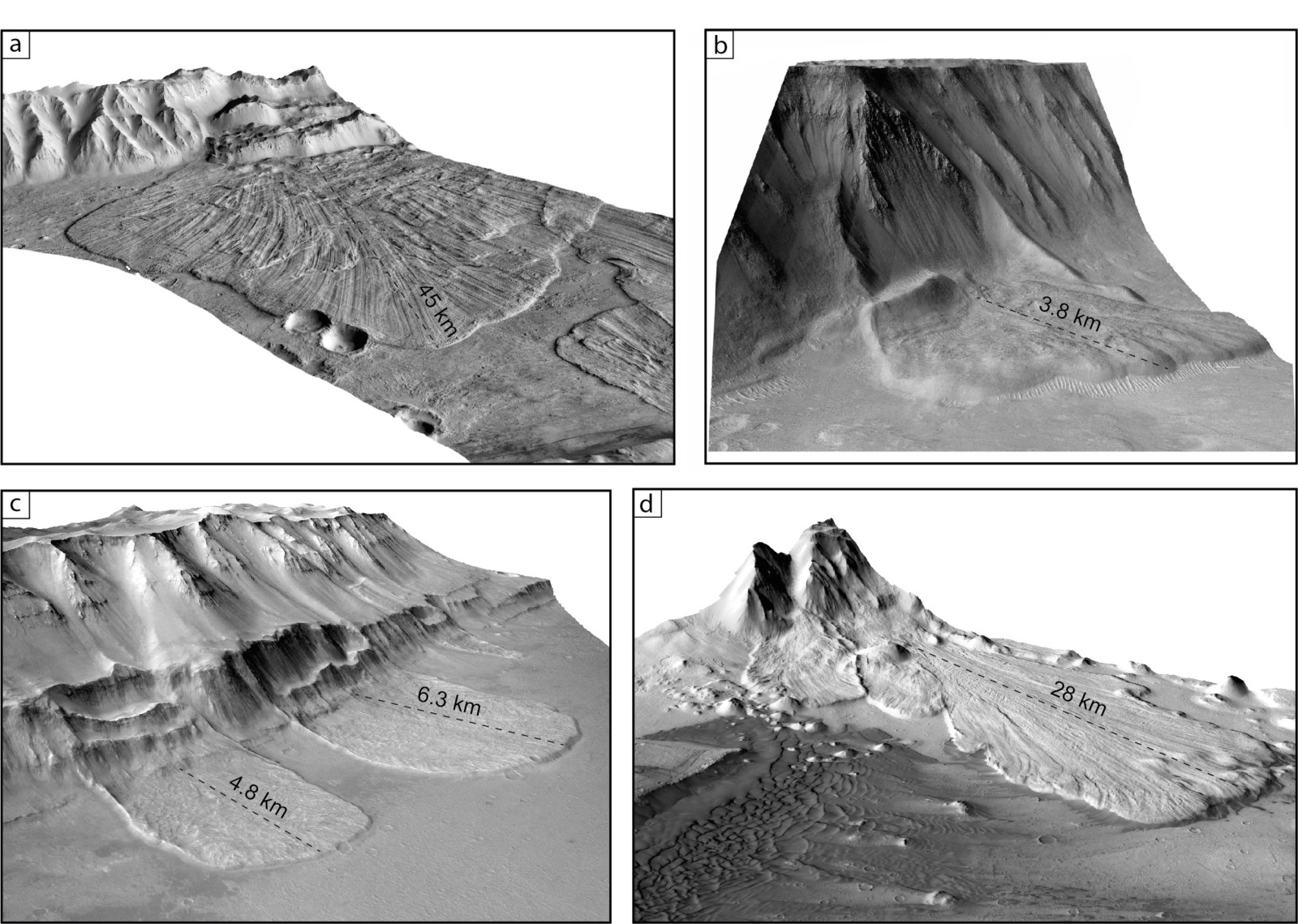
Figure 3: Oblique views of martian long runout landslides obtained by draping 6 m/px orthoimages over 20 m/px digital elevation models. All views have 1.5 vertical exaggeration. A) Coprates Labes landslide in Coprates Chasma. B) Landslide in Shalbatana valley. C) Landslides in North Aurorae Chaos. D) Landslide in Ganges Chasma. (Image credit: NASA/JPL/MSSS/NHM).
Likely we still must wait quite a long time before astronauts will visit a long runout landslide deposit on Mars. However, two astronauts have already conducted fieldwork at an extraterrestrial long runout landslide: Gene Cernan and Harrison Schmitt during the Apollo 17 mission to the Moon in 1972. And next year we will celebrate the 50th anniversary of what has been so far the last human mission to another planetary body, which, amongst many other things, brought back to Earth a core sample of the top 60 cm of a lunar long runout landslide.
References Crosta, G. B., F. V. De Blasio, and P. Frattini (2018a), Global Scale Analysis of Martian Landslide Mobility and Paleoenvironmental Clues, Journal of Geophysical Research: Planets, 123(4), 872-891, doi:10.1002/2017je005398. De Blasio, F. V. (2011), Landslides in Valles Marineris (Mars): A possible role of basal lubrication by sub-surface ice, Planet Space Sci, 59(13), 1384-1392, doi:10.1016/j.pss.2011.04.015. Dufresne, A., and T. R. Davies (2009), Longitudinal ridges in mass movement deposits, Geomorphology, 105(3-4), 171-181, doi:10.1016/j.geomorph.2008.09.009. Erismann, T. H., H. Heuberger, and E. Preuss (1977), Fused Rock of Kofels (Tyrol) - Frictionite Generated by a Landslide, Tscher Miner Petrog, 24(1-2), 67-119, doi:10.1007/Bf01081746. Forterre, Y., and O. Pouliquen (2001), Longitudinal vortices in granular flows, Phys Rev Lett, 86(26), 5886-5889, doi:10.1103/PhysRevLett.86.5886. Goren, L., E. Aharonov, and M. H. Anders (2010), The long runout of the Heart Mountain landslide: Heating, pressurization, and carbonate decomposition, J Geophys Res-Sol Ea, 115, doi:10.1029/2009jb007113. Legros, F., J. M. Cantagrel, and B. Devouard (2000), Pseudotachylyte (Frictionite) at the Base of the Arequipa Volcanic Landslide Deposit (Peru): Implications for Emplacement Mechanisms, The Journal of Geology, 108(5), 601-611, doi:10.1086/314421. Lucchitta, B. K. (1979), Landslides in Valles Marineris, Mars, J Geophys Res, 84, 8097-8113, doi:DOI 10.1029/JB084iB14p08097. Magnarini, G., T. M. Mitchell, P. M. Grindrod, L. Goren, and H. H. Schmitt (2019), Longitudinal ridges imparted by high-speed granular flow mechanisms in martian landslides, Nature Communications, 10(1), 4711, doi:10.1038/s41467-019-12734-0. Magnarini, G., Mitchell, T. M., Goren, L., Grindrod, P. M., & Browning, J. (2021). Implications of longitudinal ridges for the mechanics of ice-free long runout landslides. Earth and Planetary Science Letters, 574, 117177. https://doi.org/10.1016/j.epsl.2021.117177. Magnarini, G., Mitchell, T. M., Grindrod, P. M., Schmitt, H. H., & Petro, N. E. (2021). Scaling relationship between the wavelength of longitudinal ridges and the thickness of long runout landslides on the Moon. Journal of Geophysical Research: Planets, 126, e2021JE006922. https://doi.org/10.1029/2021JE006922. Melosh, H. J. (1979), Acoustic Fluidization - New Geologic Process, J Geophys Res, 84(Nb13), 7513-7520, doi:10.1029/JB084iB13p07513. Quantin, C., P. Allemand, N. Mangold, and C. Delacourt (2004b), Ages of Valles Marineris (Mars) landslides and implications for canyon history, Icarus, 172(2), 555-572, doi:10.1016/j.icarus.2004.06.013. Salese, F., M. Pondrelli, A. Neeseman, G. Schmidt, and G. G. Ori (2019), Geological Evidence of Planet-Wide Groundwater System on Mars, Journal of Geophysical Research: Planets, 124(2), 374-395, doi:https://doi.org/10.1029/2018JE005802. Valderrama, P., O. Roche, P. Samaniego, B. van Wyk de Vries, K. Bernard, and J. Mariño (2016), Dynamic implications of ridges on a debris avalanche deposit at Tutupaca volvano (southern Peru), Bull Volcanol, 78:14, DOI 10.1007/s00445-016-1011-x. Vardoulakis, I. (2000), Catastrophic landslides due to frictional heating of the failure plane, Mech Cohes-Frict Mat, 5(6), 443-467, doi:10.1002/1099-1484(200008)5:63.0.Co;2-W Watkins, J. A., B. L. Ehlmann, and A. Yin (2015), Long-runout landslides and the long-lasting effects of early water activity on Mars, Geology, 43(2), 107-110, doi:10.1130/G36215.1. Watkins, J. A., B. L. Ehlmann, and A. Yin (2020), Spatiotemporal evolution, mineralogical composition, and transport mechanisms of long-runout landslides in Valles Marineris, Mars, Icarus, 350, 113836, doi:https://doi.org/10.1016/j.icarus.2020.113836.