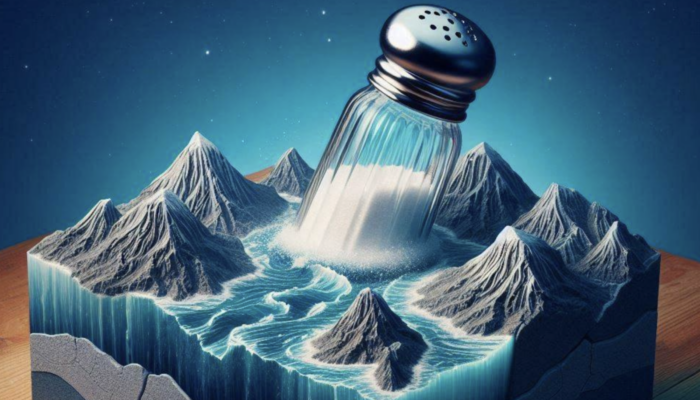
Evaporitic rocks possess unique properties that enable them to form crucial structures for petroleum systems. Salt basins are globally distributed, particularly along the Atlantic margins. Their thermal and mechanical properties can influence the Earth’s crust, altering structural styles and basin architecture, with significant implications for hydrocarbon exploration and geodynamic processes.
How different are the rocks for the geodynamicists?
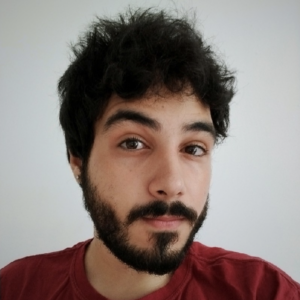
João Paulo de Souza Bueno is a Brazilian PhD student researching salt tectonics and the thermal blanket effect, with a particular focus on how it can alter the topography and temperature field of rifted margins using geodynamic models. He is also a GIS programmer and an environmental geologist with experience in geotechnical work, geological risk assessment, and mapping.
Did you consider how long we spend observing things in motion? Watching fast cars, running people, or the wind blowing the leaves in a garden? Or wondered why some materials, such as mayonnaise or peanut butter, flow when you squeeze them but hold their shape in strange ways when you stop? Thanks to scientists from ancient Greece to today, especially Navier and Stokes in the 1800s, we’ve learned a lot about rheology — the study of how materials deform and flow.
In computational geodynamics, it is common to use equations and rheological models to simulate rocks and tectonic plates as highly viscous fluids. This approach allows us to compare the results of models with what we observe in nature today. Every geodynamical model requires many parameters and conditions, such as rheological, thermal, initial velocity, and heat production. An important consideration is to think how these parameters differ between rock types, particularly the viscosity.
Viscosity is one of the most variable properties in nature. For example, at the same temperature, water has a viscosity of 1 mPa·s, while honey has a viscosity of 10,000 mPa·s. That’s why it’s easier to sip water with a straw, while it’s very difficult to sip honey. A massive jump in viscosity also occurs at the air-rock interface at the Earth’s surface, with a contrast so extreme that it’s like comparing the size of a cup of tea to the size of the Milky Way. This dramatic contrast represents one of the most significant variations in physical properties observed in nature.
When tackling geodynamic problems, the asthenospheric mantle has a viscosity of about 10²⁰ Pa·s, while the continental crust has a viscosity of 10²⁵ Pa·s—100,000 times greater (Salazar‐Mora & Sacek, 2023)! This vast difference makes viscosity one of the most important variables in geodynamic studies, as it controls the rheological behavior of all rock layers in our models.
What is salt? Is it important?
Salt (evaporitic) rocks disrupt the standard behaviour of the rocks. Crystalline and sedimentary rocks have brittle behaviour. But salt behaves very differently, with no shear strength, turning it essentially to a fluid with relatively “low” viscosity. Salt is approximately 1 million times less viscous than other rocks in sedimentary basins (Pichel et al., 2023). This incredible characteristic allows salt to flow easily, a process known as halokinesis (or salt tectonics). This movement creates unique and strange patterns and structures, deforming the surrounding sedimentary rocks in the basin.
Evaporites form when ions precipitate from brine due to evaporation, typically in hot, isolated environments such as lakes or seawater ponds (Nichols, 2010). Their composition is determined by the ions dissolved in water, with common minerals including gypsum (CaSO₄·2H₂O), anhydrite (CaSO₄), and halite (NaCl). The evaporation process can last for hundreds of thousands of years, leading to the formation of salt layers above other rock layers.
Due to its high impermeability and irregular shape, salt rocks create great traps for hydrocarbons, making them important parts of petroleum systems. As a result, seismic surveys play an essential role in helping geologists and geophysicists to identify the most promising locations for drill holes and the placement of exploratory wells, potentially saving millions of dollars per operation.
A well-known and extensively studied region of salt tectonics is the Atlantic salt basins! These basins, located along the eastern coast of Brazil and western coast of Africa, are the main source of oil and gas of the countries in this region. After years of surveys and a lot of invested money, researchers have gained valuable insights into how salt behaves and influences the sediment dynamics in these basins. The global distribution of salt basins is detailed in Hudec & Jackson (2007).
The structures formed by salt flow are generally referred to as diapirs. For much of the last century, the main hypothesis behind diapirism was that the flow was primarily driven by buoyancy, like in a lava lamp. Today we know that the main mechanism behind the positioning and shape of the salt bodies is differential loading.
A good example of diapir growth can be demonstrated with some everyday materials: sand and silicone (Figure 1). Guglielmo et al. (1995) conducted this educational experiment applying extension to horizontal layers of sand (simulating brittle rocks) that covered a silicone layer (simulating salt). The applied extension created a graben in the overburden sand in the center of the model, thinning the layers. The resulting faults reduce the sand strength, and the thicker portion at the edges “squeeze” the silicone, causing diapirism.
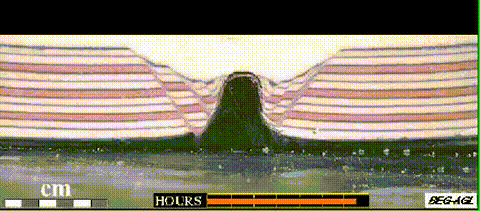
Figure 1: Experiment made by Guglielmo et al. (1995). Sand layers are in white and red. It is possible to see the analogous salt layer (black) rising and growing by differential loading, formed by extension.
The diapirism can create several structures (Figure 2), causing salt layers to rise and deform the surrounding region, which in turn creates new accommodation space (minibasins). This leads to the thickening of the sedimentary layer and enhances the thermal blanket effect, potentially affecting the thinned crust below. Formed millions of years ago, these layers and structures play a crucial role in our modern economy, trapping oil and gas in reservoirs (Fossen, 2016).
A classic example of a salt basin is found in southern Brazil, with similar basins located along the West African margin. Figure 3 shows a typical interpretation of the Santos-Benguela margin, where the Aptian/Albian salt layer is visibly deformed and pierces the overlying layers. The salt, originally deposited in the late Aptian (Ariri Formation in the Santos Basin), reached thicknesses of up to 3 km (Araujo et al., 2023; Chagas et al., 2024). In some regions, post-rift sediments directly overlay the pre-rift sequence. Additionally, the diapir patterns vary significantly across the margin: in the inner proximal domain, salt domes can reach heights of up to 8 km, contrasting with the patterns observed in other domains.
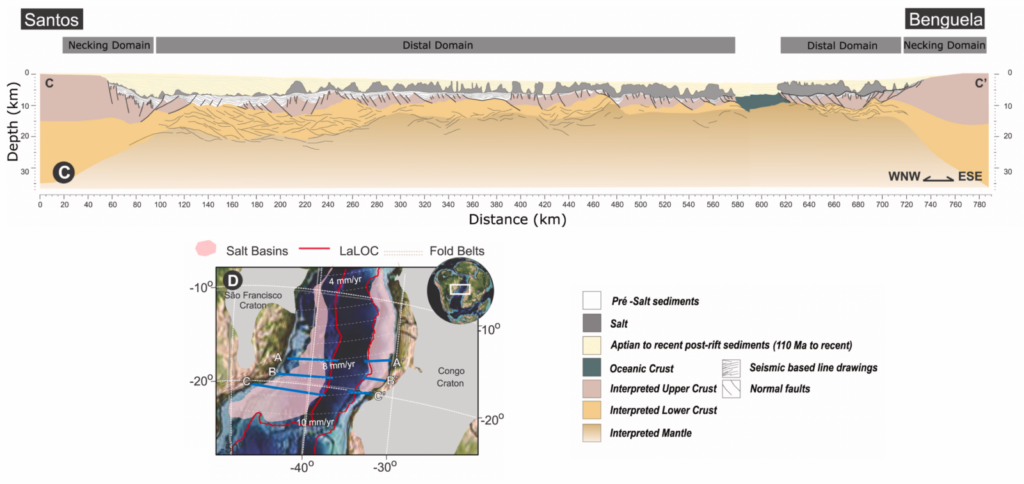
Figure 3: Santos-Benguela interpreted margin in central portion of Central Atlantic. It is possible to see the salt expulsion to distal domains. Modified from Dos Santos Souza et al. (2025).
In contractional regimes, such as orogenic wedges, salt plays an important role as a décollement layer, a layer that allows the decoupling of the overlying layers from the substrate. This effect potentially influences how strain is accommodated, enabling a highly folded rock unit to be separated from a less deformed overburden, for example (Fossen, 2016). As a result, salt facilitates thin-skinned tectonics in collision zones, such as the foreland regions (Figure 4).
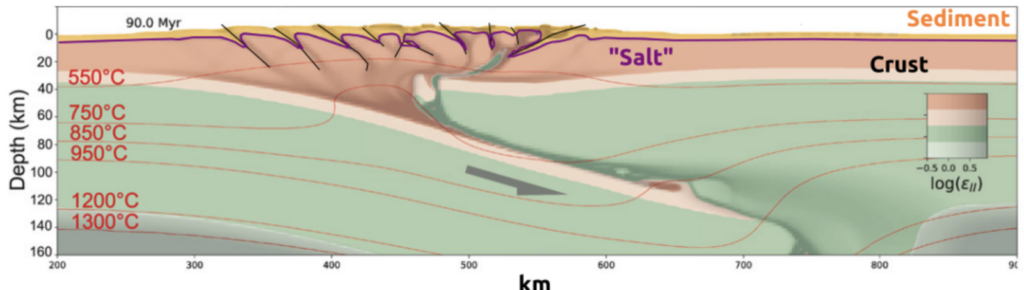
Figure 4: Example of salt acting as a décollement, decoupling the basement from the contracted sedimentary cover. Modified from Salazar-Mora et al. (2023).
Salt and geodynamics: models and implications
Salt has a density about 2,200 kg/m³ while the crust density is equal to 2,700 kg/m³. However, the porosity of sediments can reduce this value to about 1,800 kg/m³. Recent geodynamic models have shown that halokinesis becomes more intense when carbonate precipitation occurs in minibasins. Carbonate loads are heavier than clastic sediments, causing diapirs to grow taller and become more irregular (Figure 5). This process expands minibasins and leads to salt inflation (Pichel et al., 2023). This process can impact local crustal isostasy, which in turn influences the structural style of the overlying sediment package.
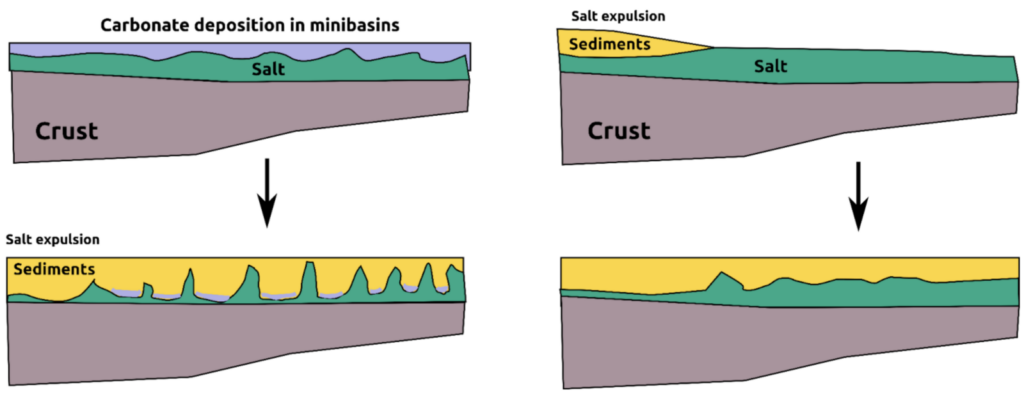
Figure 5: Schematic diagrams illustrating changes in salt structure due to carbonate deposition. Left: with dense carbonate deposition; right: without carbonate deposition, prior to clastic progradation.
The thermal effect of salt is particularly curious. Salt exhibits a thermal diffusivity of approximately 6·10-6 m²/s (varying with composition), which is six times greater than of most rocks, while sediments typically have a thermal diffusivity of 0.7·10-6 m²/s . This makes salt an exceptionally efficient thermal conductor among sedimentary rocks! As a result, it creates thermal anomalies in the basin, with diapirs transferring heat toward the surface and into the overlying layers. This process can slow down oil maturation beneath the salt (Cedeño et al., 2019).
Additionally, since temperature affects viscosity and rheological behaviour, the temperature anomalies caused by diapirs can influence these properties, altering the stress applied to the rocks, and controlling the evolution of the basin! To examine the specific thermal effect of salt rocks, I ran two sets of models (displayed in Figure 6): one with constant thermal diffusivity and another with variable thermal diffusivity for layers that represent the basin. The geometry was simplified to a 40 km thick crust, a 3 km thick salt layer, and a 6 km thick sedimentary layer.
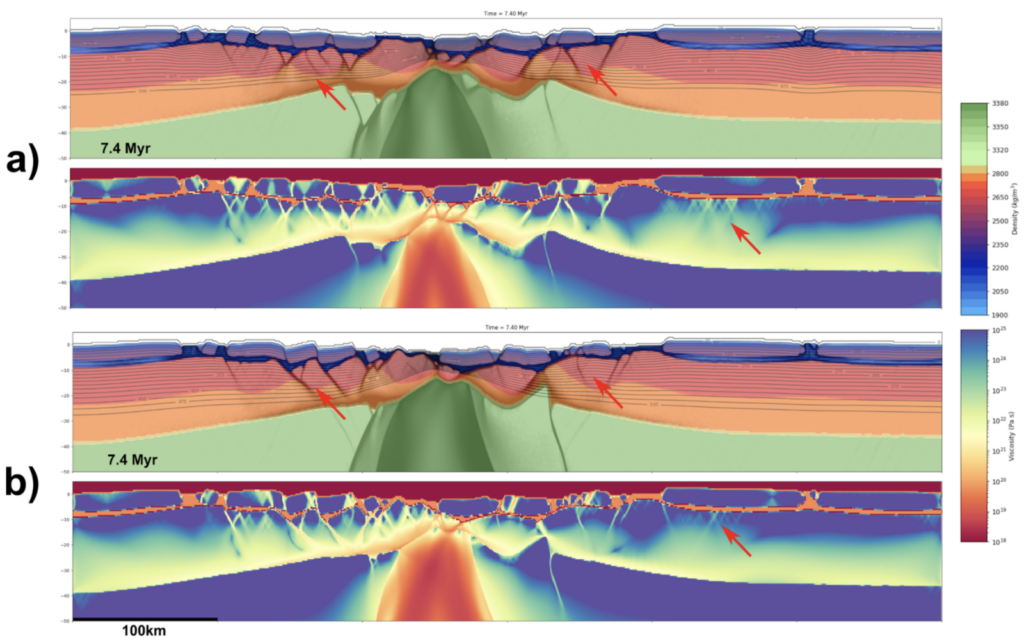
Figure 6: a) Model with variable thermal diffusibility, showing distributed faults in the left margin and a more brittle regime in the right margin. b) Model with constant thermal diffusibility, showing a more brittle domain and a significant difference between the blocks in the left and right margins.
The models started with horizontal layers subjected to extensional stress, simulating a rift with a spreading rate of 2 cm/year. A partial result (Figure 6) provides evidence that a salt layer can alter crustal architecture during rifting. The isotherms are clearly bent around the diapirs, and the analysis shows that heat diffusion is more pronounced in larger diapirs. In areas with salt, temperature variations can reach up to 100°C between different scenarios. Another interesting rheological effect is the difference in fault development (indicated by red arrows). In Scenario A, more faults form but with less strain, while Scenario B exhibits a more brittle behavior, with fewer faults but higher strain.
In conclusion, salt rocks play a crucial role in the formation of structures important within petroleum systems. Their unique thermal and mechanical properties not only influence the Earth’s crust but also shape the structural evolution and architecture of basins. Understanding these properties is essential for hydrocarbon exploration, and they directly impact the development of reservoirs and the broader geodynamic processes at play.
References:
[1] Araujo, M. N., Pérez-Gussinyé, M., & Muldashev, I. (2023). Oceanward rift migration during formation of Santos–Benguela ultra-wide rifted margins. Geological Society, London, Special Publications, 524(1), 65–91. https://doi.org/10.1144/SP524-2021-123
[2] Cedeño, A., Rojo, L. A., Cardozo, N., Centeno, L., & Escalona, A. (2019). The Impact of Salt Tectonics on the Thermal Evolution and the Petroleum System of Confined Rift Basins: Insights from Basin Modeling of the Nordkapp Basin, Norwegian Barents Sea. Geosciences, 9(7), 316. https://doi.org/10.3390/geosciences9070316
[3] Chagas, A. A. P., Araújo, C. C. de, & Santos, L. A. (2024). As grandes descobertas do pré-sal no Atlântico Sul. Petrobras.
[4] Fossen, H. (2016). Structural geology (Second edition). Cambridge University Press.
[5] Hudec, M. R., & Jackson, M. P. A. (2007). Terra infirma: Understanding salt tectonics. Earth-Science Reviews, 82(1–2), 1–28. https://doi.org/10.1016/j.earscirev.2007.01.001
[6] Nichols, G. (2010). Sedimentology and stratigraphy (2. ed., [Nachdr.]). Wiley-Blackwell.
[7] Pichel, L. M., Huismans, R. S., Gawthorpe, R., & Faleide, J. I. (2023). Post-salt carbonates control salt-tectonic minibasin formation. Geology. https://doi.org/10.1130/G51717.1
[8] Salazar‐Mora, C. A., & Sacek, V. (2023). Effects of Tectonic Quiescence Between Orogeny and Rifting. Tectonics, 42(2), e2022TC007492. https://doi.org/10.1029/2022TC007492
[9] Guglielmo, G., Jr., Vendeville, B. C., Schultz-Ela, D. D., and Jackson, M. P. A., 1995, Animations of salt diapirs, Part I: AGL95-MM-001. A BEG hypertext multimedia publication in the Internet at: https://www.beg.utexas.edu/agl/animations/AGL95-MM-001.