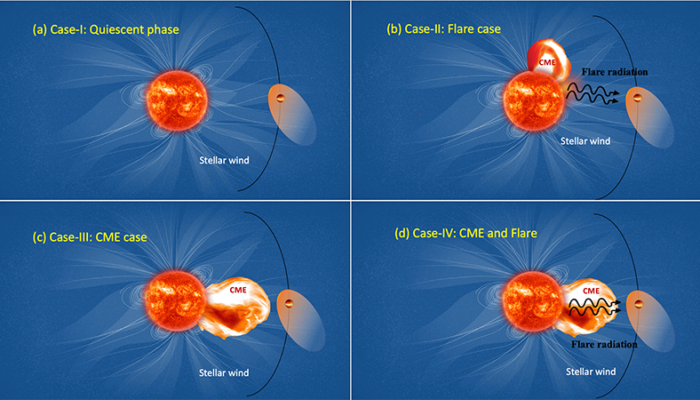
Since the discovery of the first planet around a solar like star outside of our solar system in 1995 Mayor & Queloz (1995), more than 4000 exoplanets are found so far orbiting other stars. In the recent years, the study of planets outside of our solar system i.e., extrasolar planets or exoplanets has become a very diverse and dynamic field of research in astronomy. We have developed new techniques to detect and characterise those planets in great detail including their composition and formation history. Study of exoplanets made us aware that instead of an orderly solar gaseous nebula origin (classical planet formation model for the solar system planets), chaos rules the formation of planetary systems. Due to observational bias, many of the discovered exoplanets are giant and found to orbit very close to their host stars. In the figure 1, the discovered planets as a function of their mass and their orbital distance from their host star are shown. As it is evident in the plot (figure 1) that most of the discovered planets are massive and of mass comparable to the Jupiter (1–10 MJup). These planets are very hot (surface temperature is in the range of 2000-3000K) for being very close to their host star ( less than 0.1 AU, one tenth of the distance between the Sun and the Earth) and so, are called hot Jupiters.
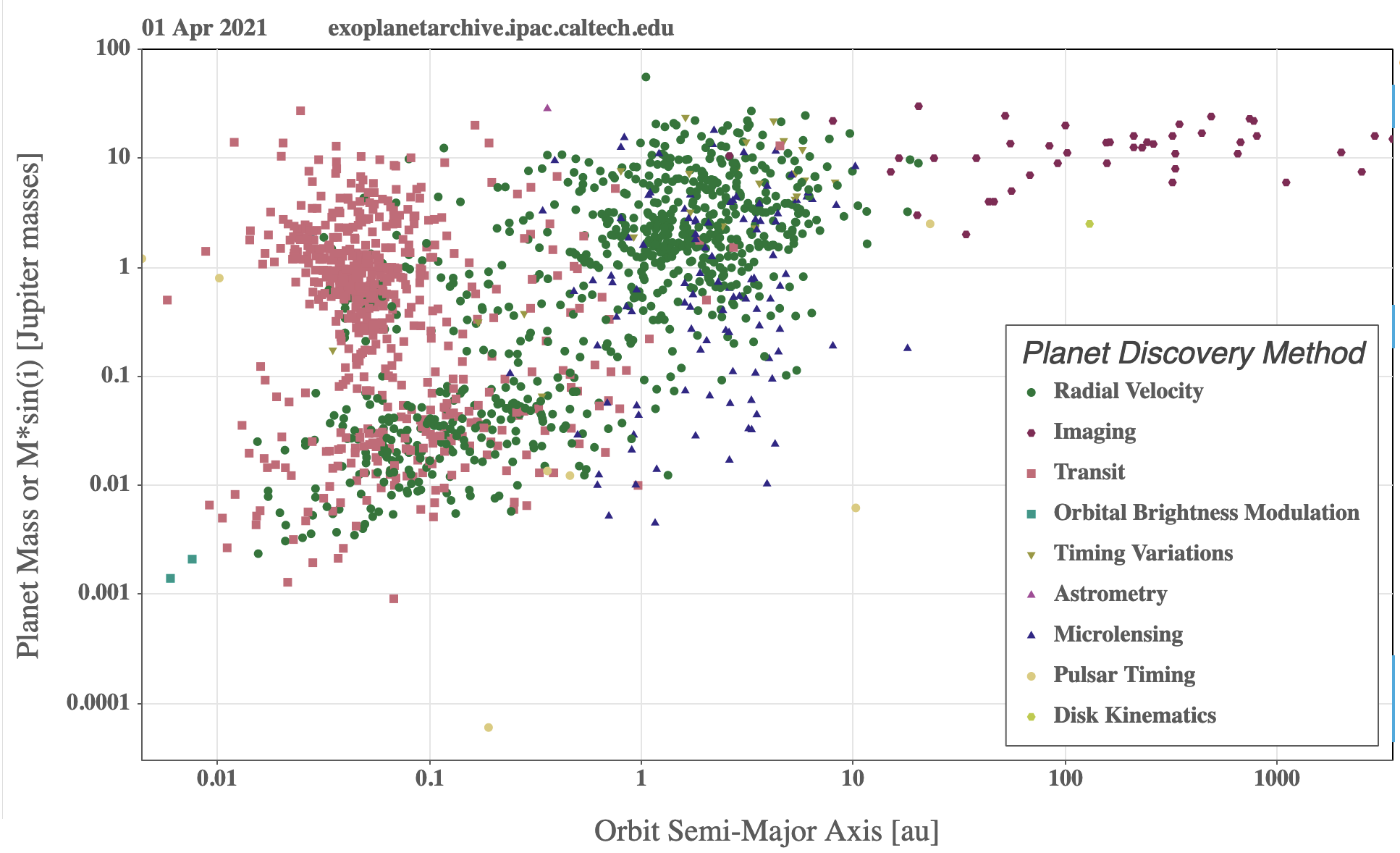
Figure 1: Mass-distance distribution of the discovered exoplanets till April, 2021
Among multiple planet detection methods, the transit method of planet detection (planets detected by the transit is shown in dark brown points in figure 1) gives us an immense opportunity to study composition of the planetary atmosphere. In figure 2, I have shown a schematic diagram of how the method works. As shown in the figure 2, when a planet transits its host star, the light from the stars passes through its atmosphere and gets absorbed depending upon its atmospheric composition. If the atmosphere of the planet is primarily dominated by hydrogen, then we can measure an absorption in the hydrogen spectrum (Lyman-α line, H-α line) due to presence of hydrogen in the planetary atmosphere. Also, by measuring the amount of the hydrogen is being absorbed by the planetary atmosphere we can determine the extension of the atmosphere, specially if the atmosphere is bound to the planetary gravity or escaping the planet.
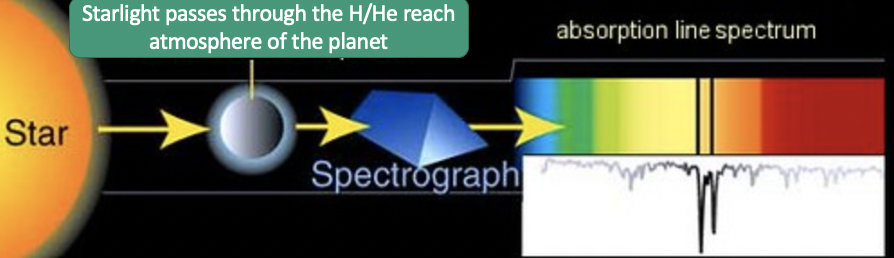
Figure 2: Schematic diagram showing the working principle of transit spectroscopy
The first observational evidence of an escaping atmosphere from an exoplanet was found by detecting the presence of atomic hydrogen beyond the Roche lobe distance (the distance up to which the planetary gravity is dominant) for the hot Jupiter HD209458b (Vidal-Madjar et al. 2003). Many subsequent observations (Vidal-Madjar et al., 2004; Linsky et al., 2010; Cubillos et al., 2020) also established the presence of atomic oxygen, ionized carbon, silicon, magnesium and ionized iron at very high altitudes from the planet making it clear that not only hydrogen but other heavier materials are also going through an atmospheric escape. Also, transit measurements over the interval of a few years showed a variation in the atmospheric escape rate from exoplanets implying a stellar origin in the difference in the planetary mass-loss rate. In particular, Lecavelier des Etangs et al. (2012) observed two transits of the hot Jupiter HD189733b on two epochs using Space Telescope Imaging Spectrograph (STIS) on board the Hubble Space Telescope (HST). While evaporation was not detected in the first epoch of observations (April, 2010), in the second epoch (September, 2011), an absorption depths of 14:4% ± 3:6 due to high-velocity planetary material coming towards the observer was observed in the Lyman-α line. During the second transit observation, an X-ray are on the host star just 8h before has been also observed supporting the idea that the atmosphere of HD189733b went through an enhancement of escape caused by a transient event of the host star (Lecavelier des Etangs et al., 2012). This is the first observational confirmation that the stellar storms i.e., coronal mass ejection (hence CMEs) and fares in the host stars are responsible for the origin in the difference of atmospheric escape rate from planets (Lecavelier des Etangs et al., 2012) and hence their evolution.
The storms in the Sun in the form of steady state solar plasma wind, coronal mass ejections (CMEs, a sudden large expulsions of plasma) and solar flares (a sudden explosion of radiation energy) are already known to affect the atmospheres of the terrestrial planets including our Earth. Similarly it is expected that the storms in the other host stars are going to affect the atmosphere of the exoplanets. In our recent work (Hazra et al., 2021), we studied how the stellar storms in the host star are affecting the atmospheres of the hot Jupiter HD189733b using numerical simulation and whether the synthetic transit spectra produced from our simulation can explain the above mentioned time variation in the transit observation of atmosphere of hot Jupiter HD189733b.
Modelling planetary outflow from exoplanets:
As the gaseous hot Jupiters orbit very close to their host stars ( ≤ 0.1 AU, for a reference the Sun-Earth distance is 1 AU) and are always exposed to intense radiation from their host stars, it is believed that stellar radiation (mostly X ray and Extreme Ultra Violate hence XUV part of the spectra) ionises the planetary material (photoionisation) and helps it to escape the planetary gravity in the form of a planetary outflow, which is known as the photoevaporation process. Recently, transit spectroscopic observations of some other targets (e.g., Wasp-12b, HD189833b and Kelt-9b) including HD189733b found that atmospheric escape via photoevaporation is likely a general phenomenon in hot Jupiters that could contribute to their evolution (Johnstone et al., 2015; Allan & Vidotto, 2019; Hazra et al., 2020). It is believed that the similar process was also responsible to completely erode the primary H/He atmosphere from the young Earth.
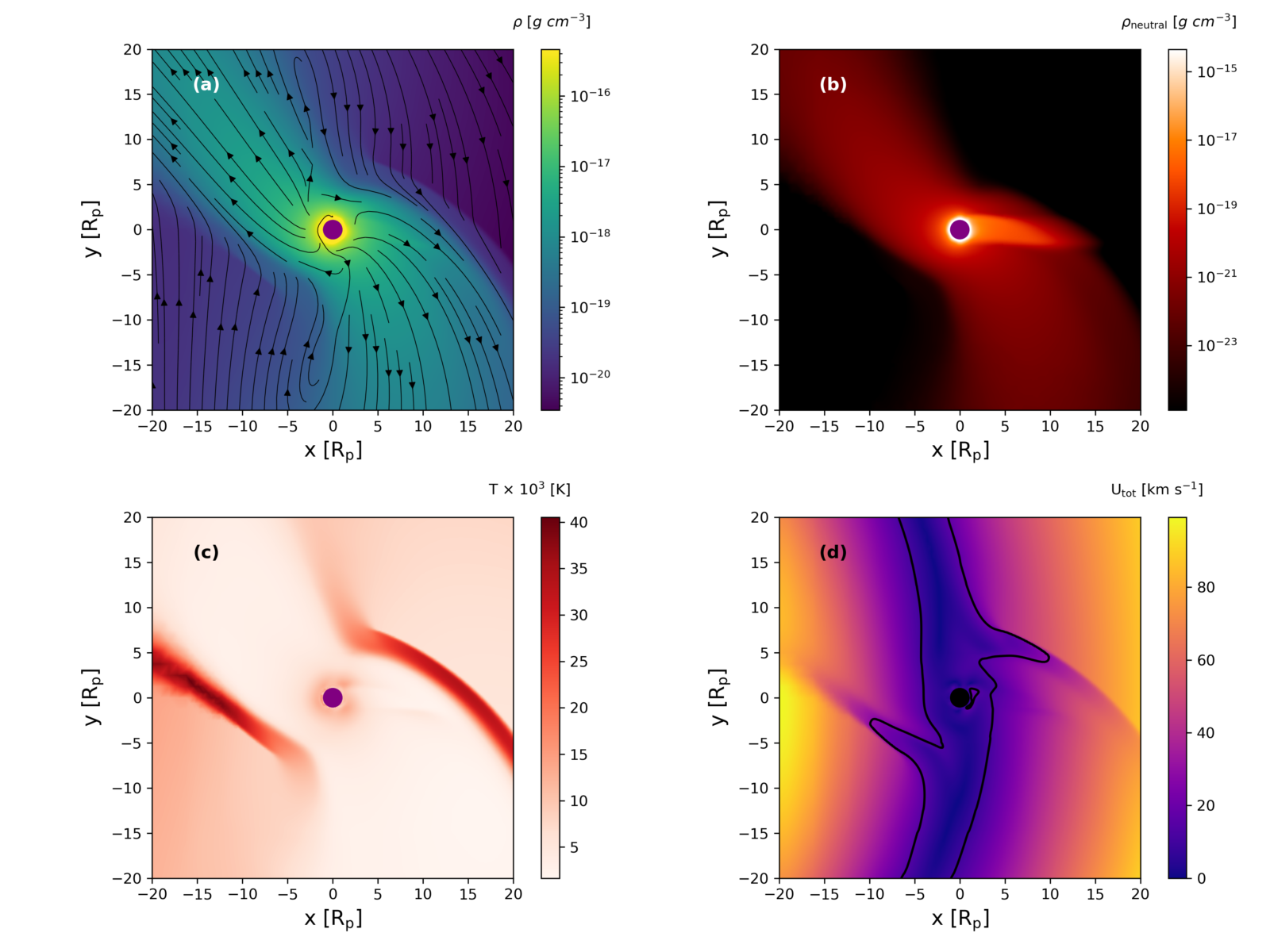
Figure 3: (a) How the total mass density of planetary material are distributed around the planet is shown along with the velocity streamlines in black lines. (b) distribution of density of neutral material. (c) Temperature distribution, and (d) Total velocity of the planetary material. Black contour shows the sonic surface where the mach number is one. The planet is shown as the solid purple circle in each panel. All plots are in the orbital plane of the planet.
In our present work, we develop a 3D radiation hydrodynamic escape model where the stellar radiation coming from the host star drives a planetary outflow self-consistently due to deposition of the stellar photons on the atmosphere of the exoplanets. We solve the mass continuity equation, momentum conservation equation and energy equation along with an ionisation equation in the rotating orbital frame of the planet. We use this model to generate the planetary outflow for the hot Jupiter HD189733b. The basic features, total density, density of neutral material (total density – density of ionised material), temperature, and total velocity of the planetary outflow are shown in figure 3 (a), (b), (c) and (d) respectively. The planetary material moves in a clockwise direction after it escapes the planetary gravity due to the Coriolis force.
Impact of storms on the exoplanetary atmosphere:
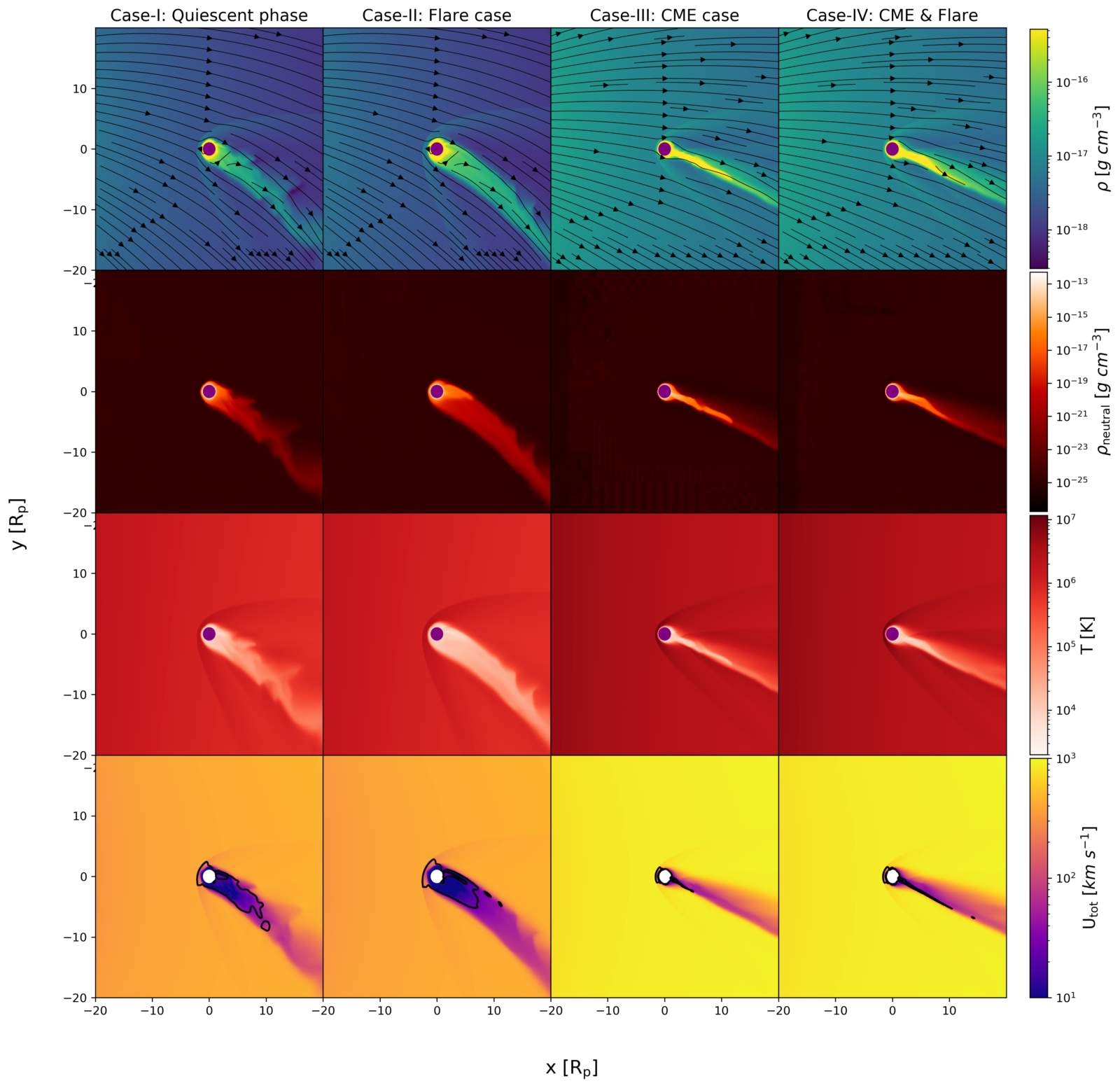
Figure 4: In the first column, the total density structure, density of neutral material, temperature, and total velocity of planetary materials are shown from top to bottom row respectively. Black streamlines in the total density plots are the velocity streamlines. Black Contours in the total velocity plots at bottom row shows the sonic surfaces. Same quantities as the first column are shown from bottom to top row for the flare case, CME case and CME \& Flare case in the second column, third column and fourth column respectively. The planet is shown in the purple solid circle except in the bottom row which is shown by a solid white circle and all the plots are in the orbital plane of the planet.
After the planetary outflow is self-consistently driven from the planet by stellar heating, we study the effect of stellar storms on its atmosphere and corresponding atmospheric evolution. We consider four cases:
Case-I: When the host star is in quiescent phase i.e., there are no storms in terms of flares or coronal mass ejections (CMEs) occurrence but the (quiescent) background stellar wind interacts with the planetary outflow
Case-II: When a flare is happened on the host star and it enhances the XUV radiation from the star. Note that, in this case, the background stellar wind is still interacting with the planetary outflow but due to the flare event, the planet receives more XUV radiation modifying the strength of the planetary outflow.
Case-III: In this case the stellar storms are assumed in the form of CME, where the planet is facing a CME alone.
Case IV: Finally, we also study a case of CME and flares together, where a CME, associated with a flare, is directed towards the planet.
The simulated atmospheric conditions for the above four cases are shown in the figure 4. The quiescent stellar wind case, are case, CME case and CME & Flare case are shown in 1st, 2nd, 3rd and 4th column respectively. In each column, the total density, neutral density, temperature and total velocity of planetary material are shown from top to bottom row. As we see in the figure 4 that the atmospheric properties after inclusion of stellar storms are completely different than case where we did not include any stellar wind or are or CME (figure 3). Basically the stellar wind from the host star interacts with the outgoing planetary out ow and creates a comet like tail structure of escaping planetary atmospheres. The different kind of host environments ( fares, CMEs or Flare & CME together) changes the planetary atmospheres differently but overall it confines the planetary atmospheres in a comet like tail structure. The escape rate for the quiescent phase, are case, CME case and CME & Flare case are 0.8×1011, 1.0×1011, 3.2×1011 and 4.0×1011 g /s respectively. The CME cases with and without a flare is most effective to strip off the atmosphere from the planet significantly. If the host star is young and active, the CME occurrence would be very frequent and that could have a stronger contribution in the evolution of the planetary atmosphere.
Constraining atmospheric mass loss by comparing numerical results with observation:
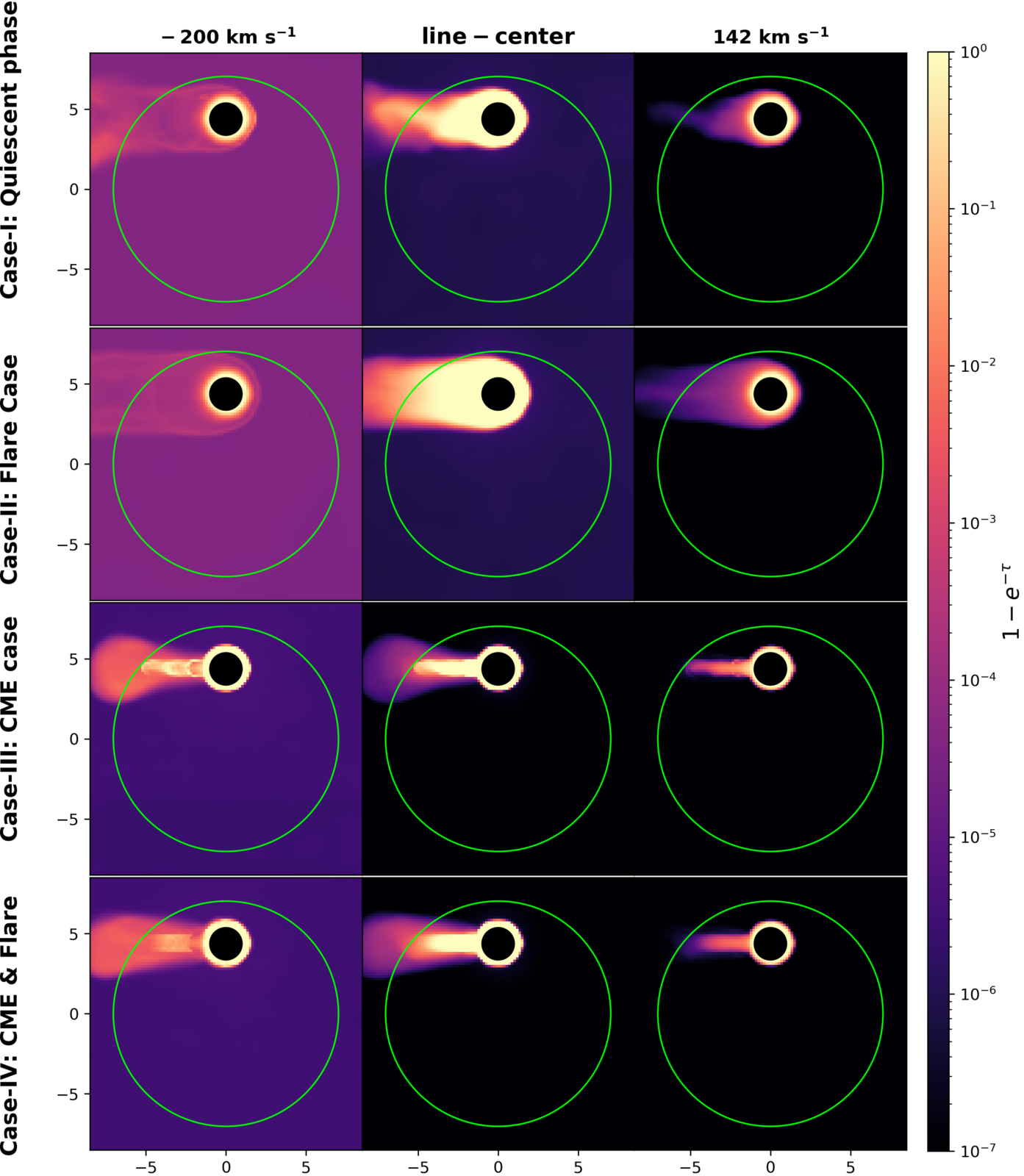
Figure 5: The left, middle and right panel show the absorbed specific stellar flux for blue shifted material at 200 km/s, at line centre and for red shifted planetary material 142 km/s respectively in the plane of the sky. Four rows from top represent the four cases from Case-I to Case-IV. The green circle is the stellar disc and solid filled black circle shows the planet.
We calculated the synthetic transit spectra in Lyman-α line for the four cases to compare with the observed Lyman-α spectra. We computed using a ray tracing code (Hazra et al., 2020) how much stellar flux is absorbed by planetary material and Figure 5 shows a view of the sky plane with the planet of absorbed stellar flux at mid-transit. The green circle represents the stellar disc. The first, second and third columns correspond to the absorbed stellar flux at three different velocities: blue shifted material at 200 km/s, at line centre and red shifted material at 142 km/s respectively. We show the transit absorption depth as a function of Doppler velocity in the figure 6 at mid-transit for the four cases along with a case where there is no stellar storm.
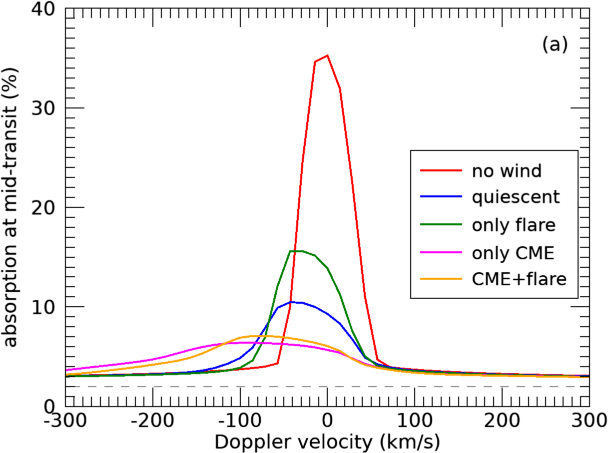
Figure 6: Theoretical Lyman-α line profiles computed at mid transit
Among all the cases considered here, Case-III (only CME) showed the highest Lyman-α absorption. The quiescent and flare cases (Case-I and Case-II) are not able to generate a strong absorption in the blue wing (i.e., high escape velocity) of the Lyman- α line. However, CME cases with and without flare (Case-IV and Case-III) show larger absorption of high velocity blue shifted material, as compared to Case-I and Case-II, as the high velocity CME carries away planetary materials with it. Therefore, the observed temporal variation in the blue wing seen in the observations (Lecavelier desEtangs et al. 2012) is more likely to be a consequence of the CME impacting the planetary atmosphere, than of the increased energy flux caused by flare. The stellar storms especially CMEs will have a long term effect on eroding the planetary atmosphere. A rough estimate of atmosphere escape by assuming the CME occurrence rate 1 per day shows that planet can lose a huge amount of its mass equal to 1.5 × 10-3 MJup via atmospheric escape. This atmospheric escape would be higher be if the strength of the CMEs is stronger and their occurrence are more frequent. The similar atmospheric escape process was also responsible to erode the primary atmospheres (H/He dominated) of the young Earth due to frequent rate of CMEs and high radiation from the young Sun.
References: Hazra G., Vidotto A. A., D'Angelo C. V., 2020, , 496, 4017 Hazra G., Vidotto Aline A. Carolan S., D'Angelo C. V., 2021, MNRAS, under review Johnstone C. P., Güdel M., Brott I., Lüftinger T., 2015, , 577, A28 Lampón M., et al., 2020, 636, A13 Lecavelier des Etangs A., et al., 2012, 543, L4 Linsky J. L., Yang H., France K., Froning C. S., Green J. C., Stocke J. T., Osterman S. N., 2010, 717, 1291 Mayor M., Queloz D., 1995, 378, 355 Vidal-Madjar A., Lecavelier des Etangs A., D_esert J. M., Ballester G. E., Ferlet R., H_ebrard G., Mayor M., 2003, 422, 143 Vidal-Madjar A., et al., 2004, 604, L69
Florian
Excellent work.