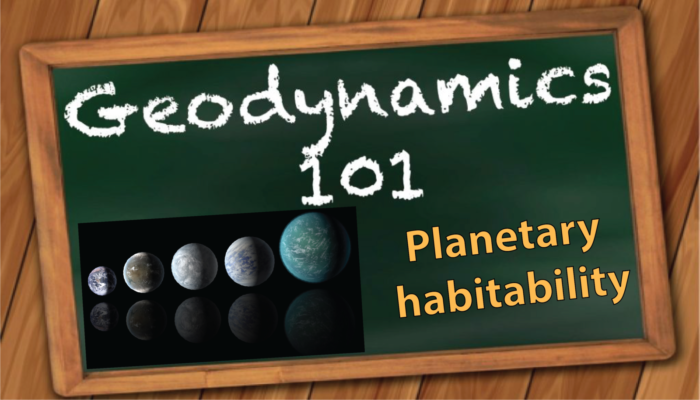
The Geodynamics 101 series serves to showcase the diversity of research topics and/or methods in the geodynamics community in an understandable manner. In this week’s Geodynamics 101 post, Brad Foley, Assistant Professor at the Department of Geosciences, Pennsylvania State University, talks about the geodynamics of planetary habitability and in particular the key role of CO2 cycling in the mantle.
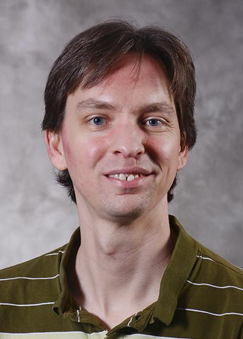
Figure 1: Assistant professor Brad Foley at the Department of Geosciences, Penn State University.
Earth’s mantle is the planet’s engine. The loss of heat from the interior to space drives Earth’s tectonic processes, mountain building and orogeny, volcanism, and the core dynamo generating Earth’s magnetic field. But perhaps less appreciated is that the mantle also plays a critical role in shaping the state of the atmosphere. This link between surface and interior evolution is not just important for studying the Earth, but also the other rocky planets in our solar system, and rocky exoplanets. Factors that make a planet, like Earth, a suitable home for life, such as the presence of liquid water oceans, weathering processes that provide critical nutrients to the oceans, and a temperate climate are all directly influenced by deep interior processes (Foley & Driscoll, 2016). Likewise, a complex interaction between life, atmospheric chemistry, weathering, volcanism, and sediment burial led to the rise of oxygen on Earth, which is both critical for some forms of life and a signature of the presence of life (Claire et al, 2006; Kump & Barley, 2007; Lyons et al, 2014). Thus, unravelling the factors that allowed Earth to develop into a planet teaming with life, whether those same factors are likely to be present on other rocky planets, and whether potential biosignatures, like atmospheric oxygen, are likely to arise on exoplanets if life is present, all require considering the role of the mantle.
Cycling of CO2: A key factor in planetary habitability
The abundance of atmospheric gases is determined by the balance between their sources and sinks, and the mantle acts as an important source and sink for many gases: volcanism releases volatiles locked in rocks to the surface, while subduction brings volatiles from the surface back into the interior. One of the most critical for habitability is CO2, which controls the climate state. On Earth, the cycling of CO2 between surface, interior, and atmosphere involve a stabilizing feedback that acts to buffer climate (Kasting & Catling, 2003). CO2 is drawn out of the atmosphere by weathering of silicate rocks and the formation of carbonate minerals on the seafloor, which are then subducted to return carbon to the mantle (Figure 2). Critically, the rate of silicate weathering increases with increasing surface temperature or atmospheric CO2. Thus when the climate warms the weathering rate increases, acting to cool the climate down, and when the climate is cool the weathering rate decreases, allowing outgassing of CO2 by volcanism to warm the climate up (Walker et al, 1981).
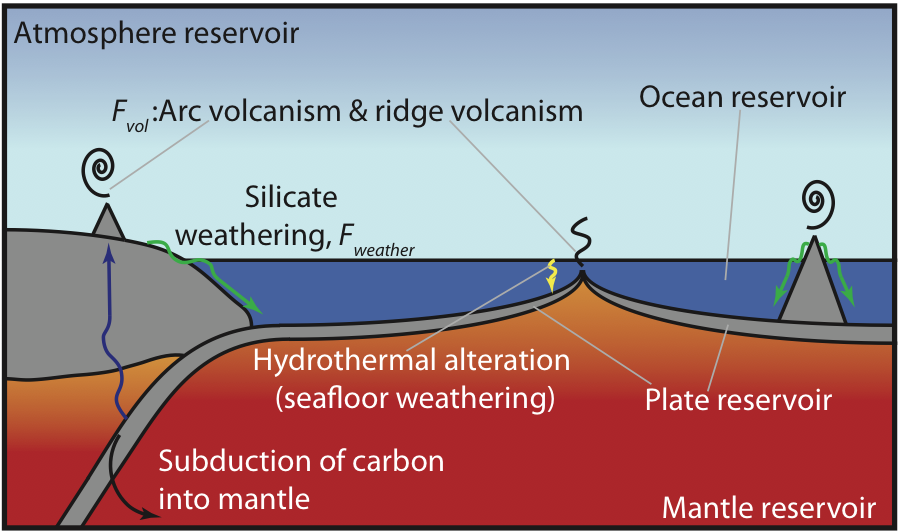
Figure 2: Schematic cartoon of the carbonate-silicate cycle on Earth. Silicate weathering on land and seafloor weathering near mid-ocean ridges remove CO2 from the atmosphere and deposit it in the ocean crust. This carbon is then subducted, where some fraction is outgassed at arc volcanoes, with the rest returning the mantle. Outgassing from mid-ocean ridges and ocean islands returns mantle carbon to the atmosphere. From Foley & Driscoll, 2016.
However, this feedback can fail in two ways: first, rates of CO2outgassing must be high enough to keep the climate from plunging into a globally glaciated or snowball state (Kadoya & Tajika, 2014); second, there must be sufficiently high rates of physical erosion to remove weathered rock and bring fresh rock into the near-surface weathering zone (Foley, 2015). The mantle plays an important role in both CO2outgassing and surface erosion rates. The CO2outgassing rate is determined by the rate of volcanism, mantle carbon content, and oxidation state, while erosion rates are controlled by rates of tectonic uplift and mountain building over geologic timescales.
Role of the mantle in CO2 cycling: Future directions
However, there are many aspects of how the mantle influences CO2 outgassing and weathering rates that are still poorly understood, and exciting avenues of future research. First-order constraints on rates of volcanism and outgassing, and how they change over time, are straightforward to calculate from both simple box models of planetary thermal evolution or 2- and 3-D mantle convection calculations (Noack et al, 2017; Tosi et al, 2017). As planets cool over time, volcanic outgassing rates decline and eventually become low enough for frozen, snowball climates to develop. Factors that keep a planet’s mantle warmer for longer, such as higher rates of radiogenic heat production or tidal heating, will thus act to prolong the lifetime of habitable surface conditions (Foley & Smye, 2018; Valencia et al, 2018). Yet there are still important uncertainties, in particular on how carbon is carried into, and circulates within, the mantle that are key avenues for future research. Moreover, the connection between mantle dynamics, mountain building, erosion, and weathering rate is still poorly understood. Erosion rates are high when topographic gradients are large, as in mountainous regions. Mountain building is most likely connected to surface plate speed and the vigor of mantle convection, however just what form this connection takes is not known. How mantle convection and plate tectonics leads to the formation of topography, and hence influences weathering and erosion, is a critical area of research for understanding the controls on long-term climate evolution.
How Earth-like must a planet be to be habitable?
Ultimately one of the most important questions driving future research in planetary evolution and exoplanets, and which geodynamicists should be a central part of answering, is how Earth-like a planet needs to be in order to sustain volatile cycles that allow for the development of life and for biosignatures, such as oxygen, to accumulate in the atmosphere once life has developed (Tasker et al, 2017). Exoplanets come in a wide range of sizes (see Figure 3): planets up to about 4 Earth masses are found to be rocky, while beyond this limit planets are volatile-rich like Neptune (Rogers, 2015), and likely compositions as well (Hinkel & Unterborn, 2018). These planets could display a range of different surface tectonic modes, including plate tectonics, stagnant lids, or some intermediary style of tectonics. Oxidation states could be different, influencing the type of gases outgassed by volcanism. Instead of outgassing predominantly CO2, a planet with a more reduced mantle could outgas mostly CO or CH4. Likewise, different crustal compositions could alter weathering processes and the stability of volatiles as they are recycled into the interior at subduction zones or by crustal foundering. Exploring these issues will require interdisciplinary research including geochemists, mineral physicists, and geodynamicists, as well as biogeochemists, climate scientists, and astronomers. With future space telescopes poised to image exoplanet atmospheres, research on the role of the planetary interior in shaping the surface environment and atmosphere has never been so relevant.
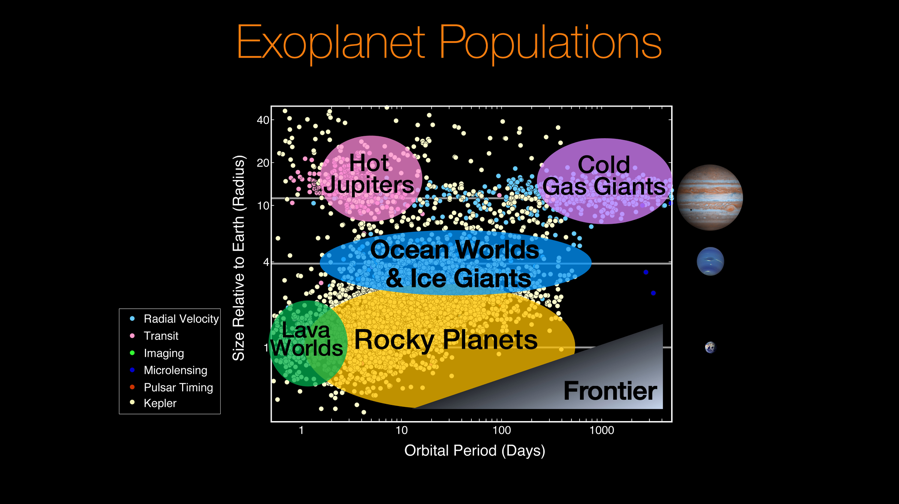
Figure 3: Exoplanet population as of August 2017. Image credit: NASA/Ames Research Center/Natalie Batalha/Wendy Stenzel
References
Claire, M. W., Catling, D. C., & Zahnle, K. J. (2006). Biogeochemical modelling of the rise in atmospheric oxygen. Geobiology, 4(4), 239-269. Foley, B. J., & Driscoll, P. E. (2016). Whole planet coupling between climate, mantle, and core: Implications for rocky planet evolution. Geochemistry, Geophysics, Geosystems, 17(5), 1885-1914. Foley, B. J., & Smye, A. J. (2018). Carbon Cycling and Habitability of Earth-Sized Stagnant Lid Planets. Astrobiology, 18(7), 873-896. Hinkel, N. R., & Unterborn, C. T. (2018). The Star–Planet Connection. I. Using Stellar Composition to Observationally Constrain Planetary Mineralogy for the 10 Closest Stars. The Astrophysical Journal, 853(1), 83. Kadoya, S., & Tajika, E. (2014). Conditions for oceans on Earth-like planets orbiting within the habitable zone: importance of volcanic CO2degassing. The Astrophysical Journal, 790(2), 107. Kasting, J. F., & Catling, D. (2003). Evolution of a habitable planet. Annual Review of Astronomy and Astrophysics, 41(1), 429-463. Kump, L. R., & Barley, M. E. (2007). Increased subaerial volcanism and the rise of atmospheric oxygen 2.5 billion years ago. Nature, 448(7157), 1033. Lyons, T. W., Reinhard, C. T., & Planavsky, N. J. (2014). The rise of oxygen in Earth’s early ocean and atmosphere. Nature, 506(7488), 307. Noack, L., Rivoldini, A., & Van Hoolst, T. (2017). Volcanism and outgassing of stagnant-lid planets: Implications for the habitable zone. Physics of the Earth and Planetary Interiors, 269, 40-57. Rogers, L. A. (2015). Most 1.6 Earth-radius planets are not rocky. The Astrophysical Journal, 801(1), 41. Tasker, E., Tan, J., Heng, K., Kane, S., Spiegel, D., Brasser, R., ... & Houser, C. (2017). The language of exoplanet ranking metrics needs to change. Nature astronomy, 1, 0042. Tosi, Nicola, Mareike Godolt, Barbara Stracke, Thomas Ruedas, John Lee Grenfell, Dennis Höning, Athanasia Nikolaou, A-C. Plesa, Doris Breuer, and Tilman Spohn. "The habitability of a stagnant-lid Earth." Astronomy & Astrophysics 605 (2017): A71. Valencia, D., Tan, V. Y. Y., & Zajac, Z. (2018). Habitability from Tidally Induced Tectonics. The Astrophysical Journal, 857(2), 106. Walker, J. C., Hays, P. B., & Kasting, J. F. (1981). A negative feedback mechanism for the long‐term stabilization of Earth's surface temperature. Journal of Geophysical Research: Oceans, 86(C10), 9776-9782.