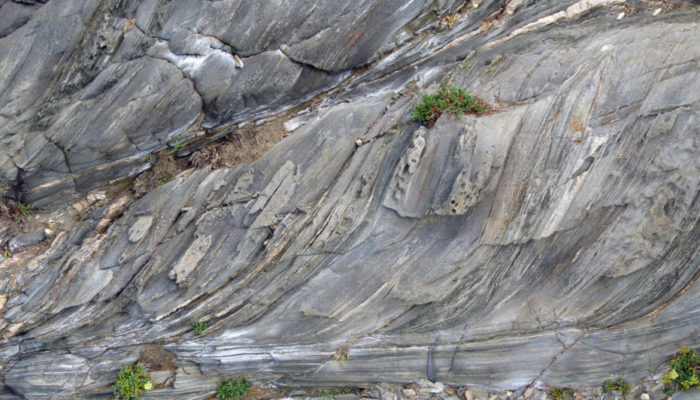
The San Andreas Fault in California, the Alpine Fault in New Zealand, or the Main Frontal Thrust in the Himalayas are some of the most famous and largest fault zones that accommodate the relative displacement between two adjacent crustal blocks.
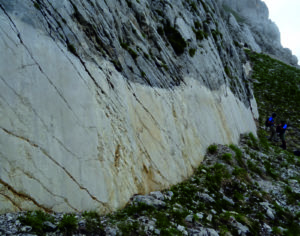
Surface rupture along the Mt. Vettore fault (“Scoglio dell’Aquila” zone) with local throw exceeding 2 meters, mainly generated after 30 October 2016 earthquake (Norcia -Italy). Faults like this one are common structures in the upper crust, where they localize seismogenic deformation. Photo © Nicola Morgantini via Imaggeo.
Such faults, however, represent only the shallower expression of something much bigger: a crustal shear zone. In the first 10 kilometers or so of the crust, rocks are cold (usually below 300 °C) and tend to fracture when subject to deformation, producing cracks and fractures that may join together to form a fault zone. Within a fault zone, deformation, which in general is accompanied by earthquakes, further fracture, crush, and pulverizes geologic materials, producing a variety of rocks such as cataclasite, gouge, and pseudotachylite (more about them in the next posts of the series). Material scientists and geologist call this behavior brittle regime, which occurs when deformation occurs by loss of cohesion of materials and strain localizes on discrete planes and structures.
But, what happens at depth? It is much hotter in the Earth’s middle and lower crust (300 – 800 °C) and there rocks behave very differently than those in the shallow crust. Hotter, deeper rocks are more similar to plastic and, at geologic timescales, they flow rather than fracture.
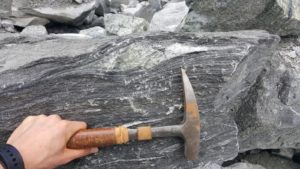
Sheared metagranodiorite from the Uchee Belt, Atlanta, Georgia (USA). Photo © mcdietel via Twitter
Wait! Rocks…flow? Yes, like most materials, higher temperature facilitates plastic deformation. Think of chocolate, for instance: it is easy to snap a chocolate square from a chocolate bar when it is cold. However, when it is hot in the summer, chocolate bends and flows, making your chocolate eating experience quite disappointing. Another example is represented by how blacksmiths shape metal: they need to heat metal up in a furnace in order to forge it. Otherwise, at low temperature, metal is quite resistant and may break during deformation.
Rocks are no different. At depth, with high temperature, they flow, shear, squeeze, and deform by crystal-plastic mechanisms, without breaking. Rocks are deformed, sheared, squeezed, and folded without fracturing and producing earthquakes. We have shown examples of this behavior – which geologists call ductile regime – in previous posts of the Features from the Field series: e.g. boudinage, foliation, and folding.
A shear zone is the ductile, deep equivalent of a fault zone. Shear zones are generally wider than faults and may accommodate displacements over a range of scales from some centimeters to tens of kilometers. Strain is considerably higher in shear zones with respect to the surrounding rocks, which may appear weakly deformed or non-deformed at all. Inside a shear zone, deformation forms spectacular well foliated, deformed and commonly lineated rocks called mylonite. The term mylonite comes from the Greek μύλος mylos, meaning ‘mill’, as early authors thought these rocks derived from crushing of the surrounding rocks. Today we know –also thanks to lab experiments– that dynamic recrystallization and metamorphic reactions instead produces the strongly deformed and fine-grained structure of mylonites.
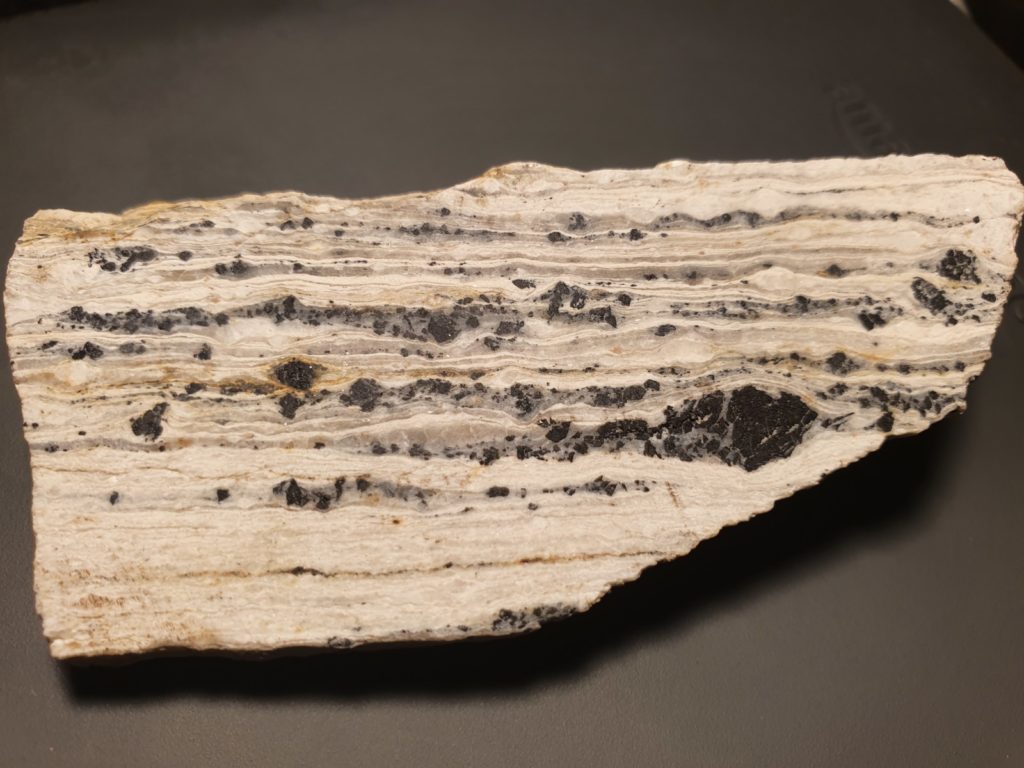
Mylonitic metagranite from the Sierra Nevada, Spain. The original minerals constituting the metagranite (grey = quartz; white: feldspar; black: tourmaline) were deformed and stretched. Tourmaline is stronger than quartz and feldspar and shows brittle behavior even in this mylonitic sample. Photo © Euan McIntosh via Twitter
Mylonites contain a series of structures that indicate ductile grain-size reduction of pre-existing rocks and may be useful to tell the direction of flow during shearing. Metagranites (i.e. deformed igneous rocks) show some of the most classical example of the development of shear zones and mylonitic structures starting from relatively intact rocks.
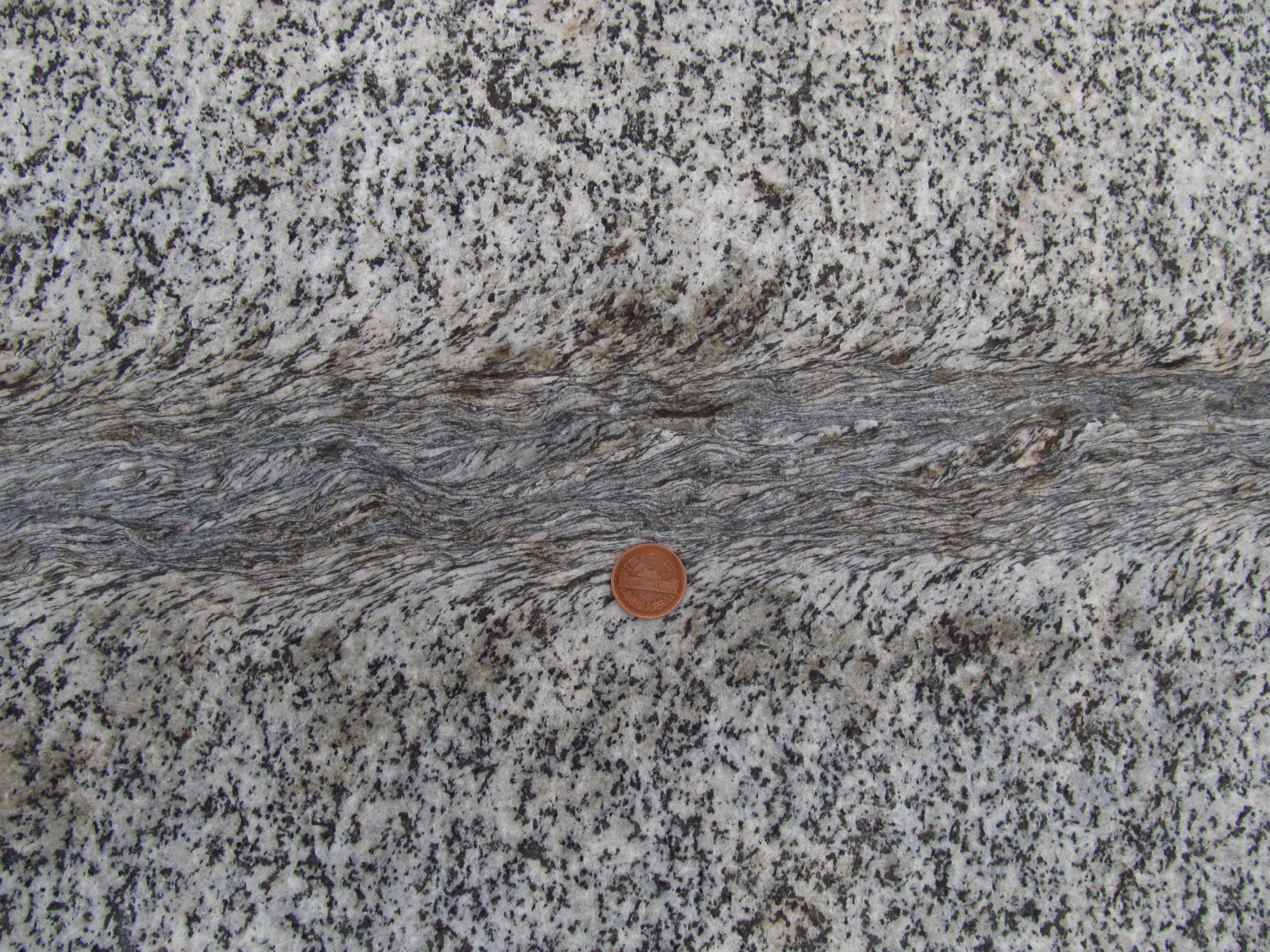
Shear zone in metagranite at the Neves Glacier (Sud-Tirol, Italy). Thanks to the organizers of the EGU Summer School for bringing me there! Photo © Samuele Papeschi
In the figure above, a horizontal shear zone crosscuts a metagranite. The difference between the host rocks and the shear zone is striking: the host rock preserves a crystalline magmatic fabric with coarse-grained igneous textured quartz, feldspar, and biotite, whereas in the shear zone shows a completely re-organized mylonitic structure. The shear zone is fine-grained, well foliated, and rich in micas with respect to the surrounding rocks. Fragments of the original igneous rock (called protolith) are present within the shear zone. If you look at the transition between the ‘undeformed’ host and the shear zone, you may notice the presence of a foliation inclined at 45° a few centimeters away from the ‘high-strain’ shear zone: approaching the shear zone itself, this foliation progressively bends and becomes parallel to it (check out also the interpreted figure, below). This structure is the result of the progressive re-organization of the igneous rock, whose grains rotate, stretch, and recrystallize as the shear zone widens. For structural geologists, this is very valuable: these features are kinematic indicators – structures that can reveal us the sense of movement of a shear zone. If you look carefully in the photo above, indeed, you can see that the drag of the foliation is consistent with the rightward displacement of the block at the top (top-to-the-right sense of shear). Because lineations here are subhorizontal, we can tell this was a dextral shear zone.
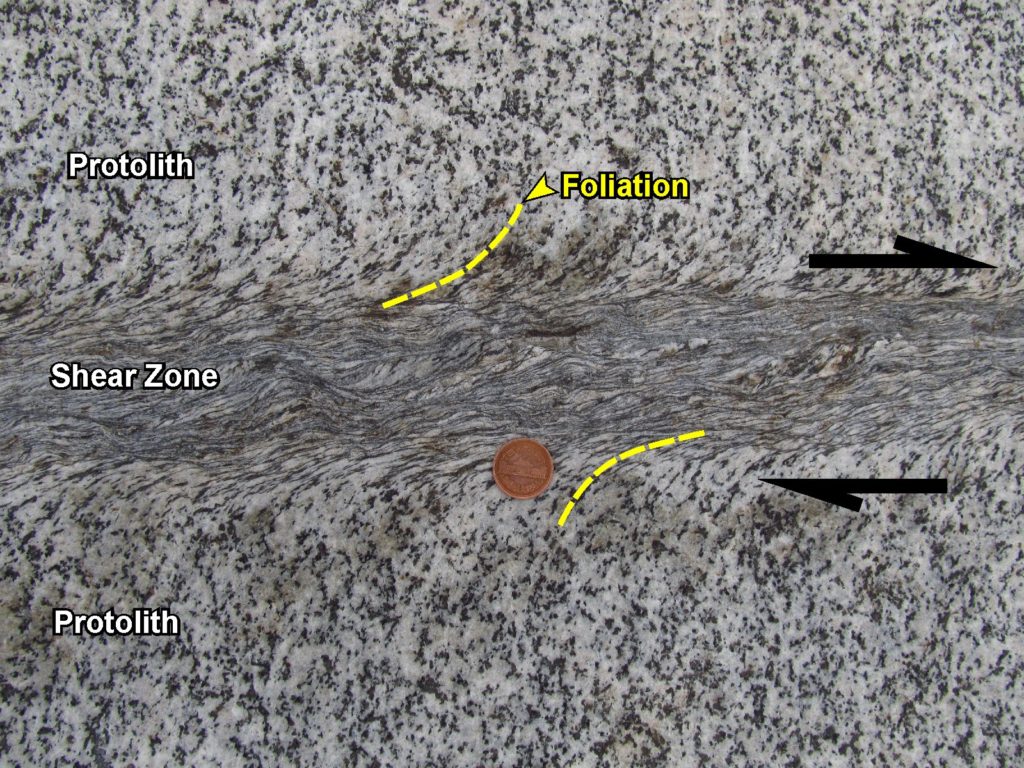
Interpretation of the Neves shear zone, shown above. The foliation (yellow) forms in the metagranite close to the shear zone and it is deflected and parallelized to the mylonitic foliation (center). In the shear zone, the metagranite has been completely transformed to a mylonite. Photo © Samuele Papeschi © Samuele Papeschi
Metagranites are wonderful, because mylonites are easily visible with respect to the surrounding igneous structures, but shear zones can nucleate in every rock – from metasediments in the crust to peridotites in the mantle – producing beautiful mylonitic structures! What changes with different rock types are the properties of the deformed grains that may exhibit brittle or ductile behavior at different temperature. For example, quartz already flows at 300 °C, while olivine can be brittle up to 700 °C.
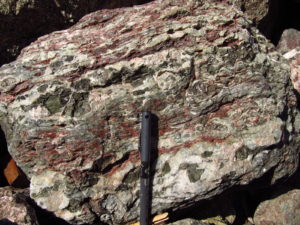
Mylonitized metagabbro from Cala del Leone (Quercianella, Italy). Unlike metagranites – that typically deform above 300 °C, metagabbros require higher temperatures (> 400 – 500 °C) in order to deform the strong plagioclase and pyroxene grains. Photo © Samuele Papeschi
Why are shear zones important?
Understanding shear zones and interpreting their deformation history represent major goals for structural geologists. Fossil shear zones, now exhumed to the surface, hold valuable clues to how mountains formed and plates collided. They are precious ‘hard drives’ of the deformation mechanisms happening at depths and temperatures that are otherwise completely inaccessible. Studying those structures helps us understand how rocks deform and how strain is distributed through the lithosphere, giving us important clues about how plate tectonics works.
Shear zones also affect our daily lives! Even if shear zone are (generally, not always) aseismic (i.e. do not produce earthquakes), they transport mechanical energy loading shallower faults that have the potential to release energy in the form of destructive earthquakes. Shear zones are also major discontinuities that may act as preferred fluid pathways through the crust, becoming potential traps for strategic metal, rare earths, and other mineralizations.
Further Reading
Sibson (1977). Fault Rocks and Fault Mechanisms.
White et al. (1980). On mylonites in Ductile Shear Zones.
Ramsay (1980). Shear zone geometry: a review.
Gapais (1989). Shear structures within deformed granites: Mechanical and thermal indicators.
Snoke et al. (1998). Fault-related Rocks: A Photographic Atlas.
Carreras (2001). Zooming on Northern Cap de Creus shear zones.
Fossen & Cavalcante (2017). Shear zones – A review.
Mountain Beltway (AGU Blogosphere)
Gallery
When I asked for field photos to write this post, I did not foresee the amount of support coming from the GEO-Twitter community! It is a pity not to show all the beautiful images that you sent to me. Therefore, I hope you will enjoy this gallery of stunning shear zone photographs!
- Set of anastomosing dextral and minor sinistral ductile shear zones across schists and pegmatite bodies. Cap de Creus (Catalunya, Spain). Photo © Elena Druguet
- Tiny, localized shear zone in metagabbro, Saxonian Granulite Massif, Germany. Photo © Bryce Carr
- Mylonized Alpujarride metacarbonate, below Veleta, Sierra Nevada (Spain). Photo © John Faithfull
- Set of anastomosing dextral and minor sinistral ductile shear zones across schists and pegmatite bodies. Cap de Creus (Catalunya, Spain) Photo © Jordi Carreras
- Strain (and foliation intensity) increases upward in this sheared migmatite from the Ivrea Zone (W Alps, Italy). Photo © Samuele Papeschi
- Dolomite (yellow) is stronger than calcite (grey) and during deformation it forms the resistant sheared layers in this mylonitic marble from the Apuan Alps (Italy). Photo © Samuele Papeschi
- Mylonitic metadolomite from the Noonday Fm., Death Valley (California). Field of view is approximately 100 cm. Photo © Vicky
- Shear zone in Archean Lewisian gneisses, near Miabhaig, Lewis. Outcrop is about 2m high. Photo © John Faithfull
- Ultramafic mylonite from the metamorphic sole of the Dinarides, Albania. Photo © Janos Urai
- Sheared Proterozoic (Iona Group) conglomerate, Uamh nan Calman, Iona (Scotland). Photo © John Faithfull
- Mylonitized metapellite from Main Central Thrust Shear zone, NW Himalaya. Photo © Shivaji Saha
- Mylonitic structures in schistose rocks from the Calamita Schists (Island of Elba, Italy). Photo © Samuele Papeschi
- Sheared amphibole-bearing granite with a sigmoidal lozenge of an intermediate intrusive rock. Tibet. Photo © Richard Palin