Glaciers are mostly made of water. Sometimes, perhaps more than we’d like, some of that water makes a break for it by melting, the inconstant molecule… It might pootle around on the surface of the glacier a bit and get a lot of remote sensers very excited, but it’s what it does once it gets to the base of the glacier that really matters for the behaviour and flow of the ice. So, in 2000 words or so, here’s an attempt to explain something that would take a term of undergraduate teaching or a whole chapter or two in a textbook. How badly can it go? Let’s see!
The Basics
So, a recap: subglacial hydrology refers to the flow of water at the base of the glacier. There is a lot of water down there – both surface water that reaches the bed through crevasses and moulins, and water produced at the base. Ice is a pretty good insulator, especially if you’ve got several hundred meters of it above you, so geothermal heat from the ground and frictional heat produced by the ice flow can get trapped at the bed and lead to melting.
This water has to go somewhere – liquid water is notable for its tendency to not stay in one place for very long. Some of it might refreeze, but, once it’s at the base, the vast majority of the water is going to end up draining in some fashion. Refreezing is more likely at the surface or inside the glacier, well before the water reaches the base – it’s just warmer at the base, so it’s harder for freezing to occur. However, when you’re underneath a glacier or an ice sheet, things aren’t quite as simple as on the surface….
Normally, water at the surface flows following the hydraulic potential gradient, which depends on gravity and the surface slope; to be even clearer: when we are at the surface water flows downhill, following the steepest slopes. However, when you’ve got a massive wodge of ice on top, this changes things. You now need to consider one more factor: pressure. Specifically, under deep ice meltwater is at immense pressure, with thicker ice exerting more pressure, because it’s heavier. In other words, the hydraulic potential gradient is now determined by a combination of the bedrock slope and the thickness of the overlying ice at that point. Depending on how ice thickness varies spatially, it is entirely possible for subglacial water to ignore gravity, at least locally, and flow uphill or parallel to the slope or pretty much in any direction, really. Though, at a large scale, it’s still going to flow downhill, towards the glacier terminus, because the bed slopes that way and the ice gets generally thinner in that direction.
The other consideration is what the water’s going to flow in. Obviously, there’s not a whole lot of space between the ice and the underlying rock or sediment. But, there is some space. And the thing about pressurised water is that it has quite a lot of erosive power. And it can also melt ice. So, some sort of subglacial drainage system can exist. The important thing to remember is that the bed of the glacier is not smooth – there are all sorts of bumps, rocks and irregularities down there – so, as ice is quite viscous, when it flows over one of them, it creates a cavity on the lee side of the bump, as it can’t deform quickly enough to close the gap. The bed of a glacier has a whole network of these cavities, which can be connected by small channels at the ice-bed interface. Consequently, a very tortuous drainage system can be created, where water will drain slowly between all these linked cavities. This is called a distributed drainage system and might be termed the default state of subglacial drainage.
Feel the Pressure
The interesting bit (for a given value of ‘interesting’. Though, if you’ve got this far, you presumably haven’t died of boredom yet) is what happens when you add more water to this system. There are two forces acting on the cavities and other spaces at the bed: ice creep (the deformation of the ice under its own weight) acting to close them and melting from the water contained in the cavities to expand them. In a distributed drainage system, cavities and channels are rather small, meaning that such a system can store a limited amount of water. This means that the melting capacity of this water is limited and ice creep will always dominate over melt-driven expansion. So, if you add water to the system, the water pressure rises, because the system can’t grow to accommodate it. If you keep adding water, at a certain point there will be enough water in the larger cavities that melt-driven expansion will start to outweigh creep closure. Larger cavities will rapidly expand and form large, efficient channels, which can store larger amounts of water and will further grow because of melting; have you ever heard of a positive feedback? Therefore, adding more water to them lowers the water pressure because channels and cavities become larger. Once these channels start forming, therefore, they start sucking in water from the surrounding higher-pressure bed areas, which only makes them grow further. The final result is that the inefficient distributed system is replaced by an efficient channelised system, where water flow may be over an order of magnitude faster. This can happen in as little as a couple of days on a small glacier, forming an extensive arborescent drainage network.
This transition from distributed to channelised drainage is what we’re pretty certain happens on mountain glaciers the world over, as the melt season kicks in and the amount of subglacial water increases. It also seems to happen on the Greenland Ice Sheet, at least, at its margins, though not in Antarctica – simply because there, even in summer, it’s still bloody cold and the amount of ice that melts is tiny. The reverse transformation happens at the end of the melt season, when subglacial water discharges drop to the extent that they no longer suffice to keep the channels open, and ice creep over the winter closes them up again. This does not mean, however, that the subglacial drainage system shuts down completely over the winter – meltwater is still being produced by frictional and geothermal heat at the base – but widespread channelisation does not survive and the system returns to its distributed state.
Getting things moving
This is all very nice, you might be thinking, but why does it matter? Because it has a major impact on the motion of the overlying ice (and also the downstream water supply, if you’re reliant on meltwater from glaciers). A key determinant of glacier velocity is the effective pressure, which is the ice overburden pressure (the weight of the overlying ice, basically) minus the basal water pressure. Essentially, it’s a measure of how in contact with its bed the ice is. A rise in basal water pressure lowers basal friction, speeding the ice up. So, distributed drainage systems, with their higher water pressures, tend to promote faster flow; lower-pressure channelised systems slow things down. And, of course, during the transition from distributed to channelised systems, when very high water pressures are reached, there can be a spike in ice velocity. Considering that on glaciers melting is driven by seasonal processes, you can see that subglacial dynamics, which is primarily driven by the availability of water, will also follow the seasonal cycle, and therefore so too will ice velocity. So, the behavior of the whole glacier directly depends on the subglacial hydrology!
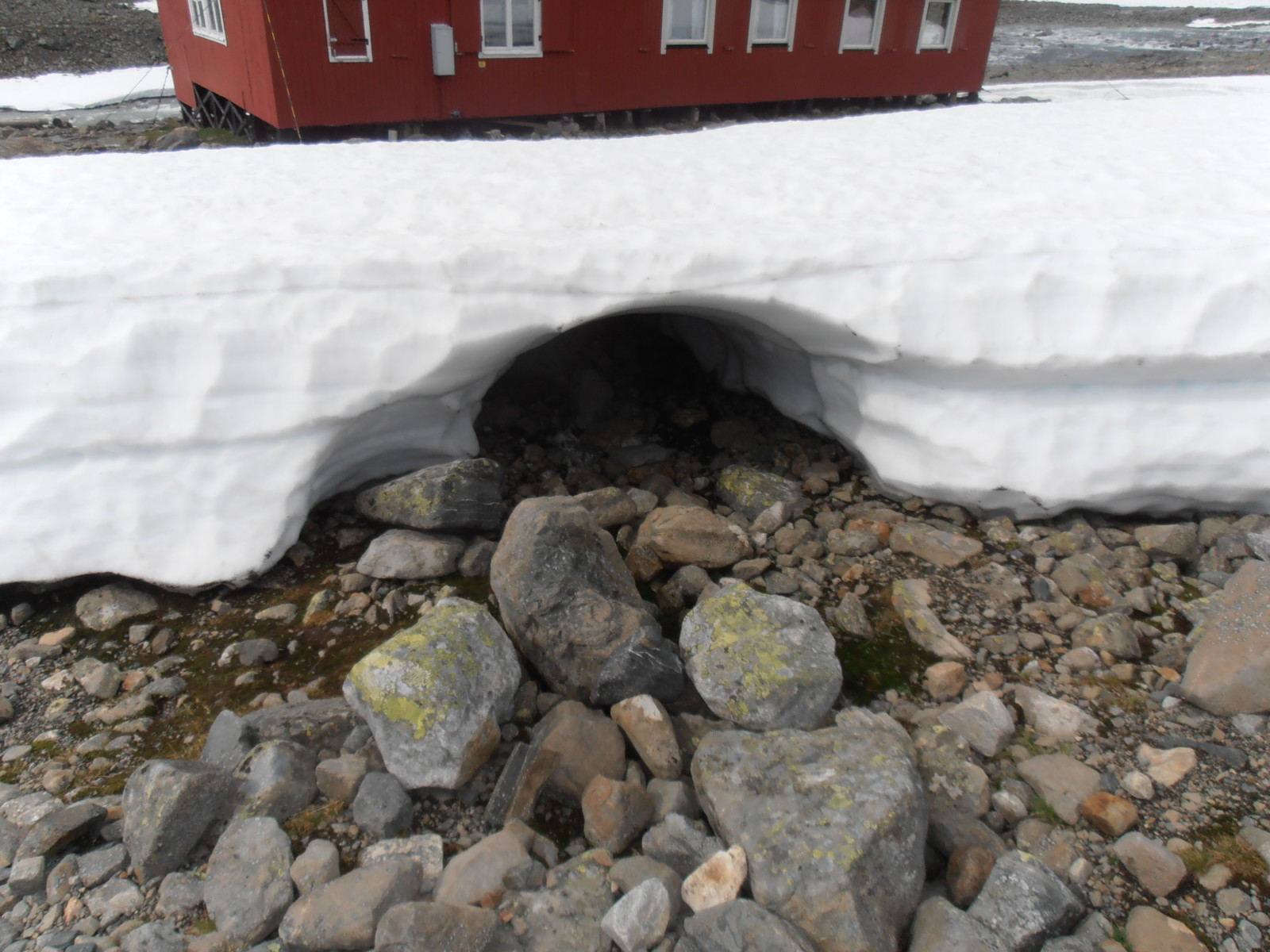
OK, this is a snowbank, not a glacier, but that gives you some idea of what the morphology of a subglacial channel might look like. Credit: Samuel Cook.
Turning things up to 11
That’s the basic idea anyway, and works pretty well on small Alpine glaciers where the theory was largely developed. Now, let’s think about that in the context of tidewater outlet glaciers in Greenland to make things more complicated and hit the advanced level. These are the way the interior ice sheet drains into the ocean. Therefore, they’re quite big – often several kilometres across and several hundred meters to well over a kilometre thick. They also flow very fast, by glacial standards, reaching speeds of several kilometers per year towards the terminus. The current record, incidentally, is held by Jakobshavn Isbrae, Greenland’s largest outlet glacier, whose terminus has been reaching the 20 km/year mark. In human terms, that makes it equivalent to Usain Bolt. On steroids. With a Falcon Heavy strapped to his back. That metaphor also works, because the long-term survival prospects of the glacier and Usain Bolt would be approximately equal, i.e. as close to 0 as makes no difference. But anyway, what does this mean for their hydrology?
Well, fairly obviously, it makes channel formation far more difficult. If the ice is thick and moving fast, you have a higher creep closure rate since new ice is continuously advected, hampering the formation of large cavities and eventually channels. Because of this, probably, tidewater glaciers don’t see the same winter/summer cycle in their hydrology as smaller glaciers, which is borne out by the fact that they flow rapidly all the time. Which is probably a consequence of them maintaining a fairly high-pressure drainage system throughout the year. Which is prevented from evolving to a low-pressure state by them flowing fast. Which is generated by the high pressure. As you can see, this is quite a circular argument – tidewater glaciers flow fast because they have a high-pressure drainage system because they flow fast. Not that there’s much that can be done to resolve this circularity, because one thing we don’t really understand yet (in glaciology, this is a very large category of things. Nearly congruent with the universal set of all things in glaciology) is how the fast ice flow that leads to these outlet glaciers initiates.
The other big difference at tidewater glaciers is that all this fresh meltwater comes out somewhere at the bottom of a salty fjord. Freshwater being less dense than saltwater, this means you get rising plumes of freshwater at the ice-ocean interface, which cause a lot of melting of the ice, because they much more effectively transfer heat from the ‘relatively warm’ water (by which I mean a whole 2°C, maybe. Balmy!) in the fjord to the ice. Are these more active when there’s more melt? Probably. Do they matter for the stability of the glacier? Possibly. Can we study them well? Ummmm….
We have a problem
I say this, because one thing people haven’t studied too much is the near-terminus hydrology of tidewater outlet glaciers; either the subglacial drainage system itself or the resulting plumes. Because it’s incredibly difficult. The usual way glaciologists find out about the structure of subglacial drainage systems is by chucking a load of dye into any available moulins and waiting to see where it comes out at the front of the glacier. The length of time it takes the dye to reach the front, how dispersed it is and the location of its efflux can tell you quite a lot about how water’s flowing under the glacier. This all goes out the window on tidewater glaciers, because, fairly self-evidently, the exit point for the water is somewhere at the base of the calving front, several hundred metres beneath the surface of the fjord. You could try to stick some detectors at the base of the calving front, but if you can work out a way to do that without dying or without having the detectors break within a day, you’ll have solved one of the big problems in glaciology. Think about it – you can’t sail up to the calving front – a) because there’s loads of icebergs and sea ice in the way most of the time and b) if you do, and stay there for any length of time, there’s a good chance an iceberg will calve on your head, or near enough that the boat will get sunk by the resulting tsunami. You could try to use some sort of autonomous or remotely-operated vehicle, but, if you did manage to get one to the front and position some detectors, the likelihood is that they’d be crushed in fairly short order by the movement of ice and icebergs. And all that’s assuming you could even deliver the dye safely – the lower reaches of these glaciers tend to be really really crevassed and not the safest place to walk…
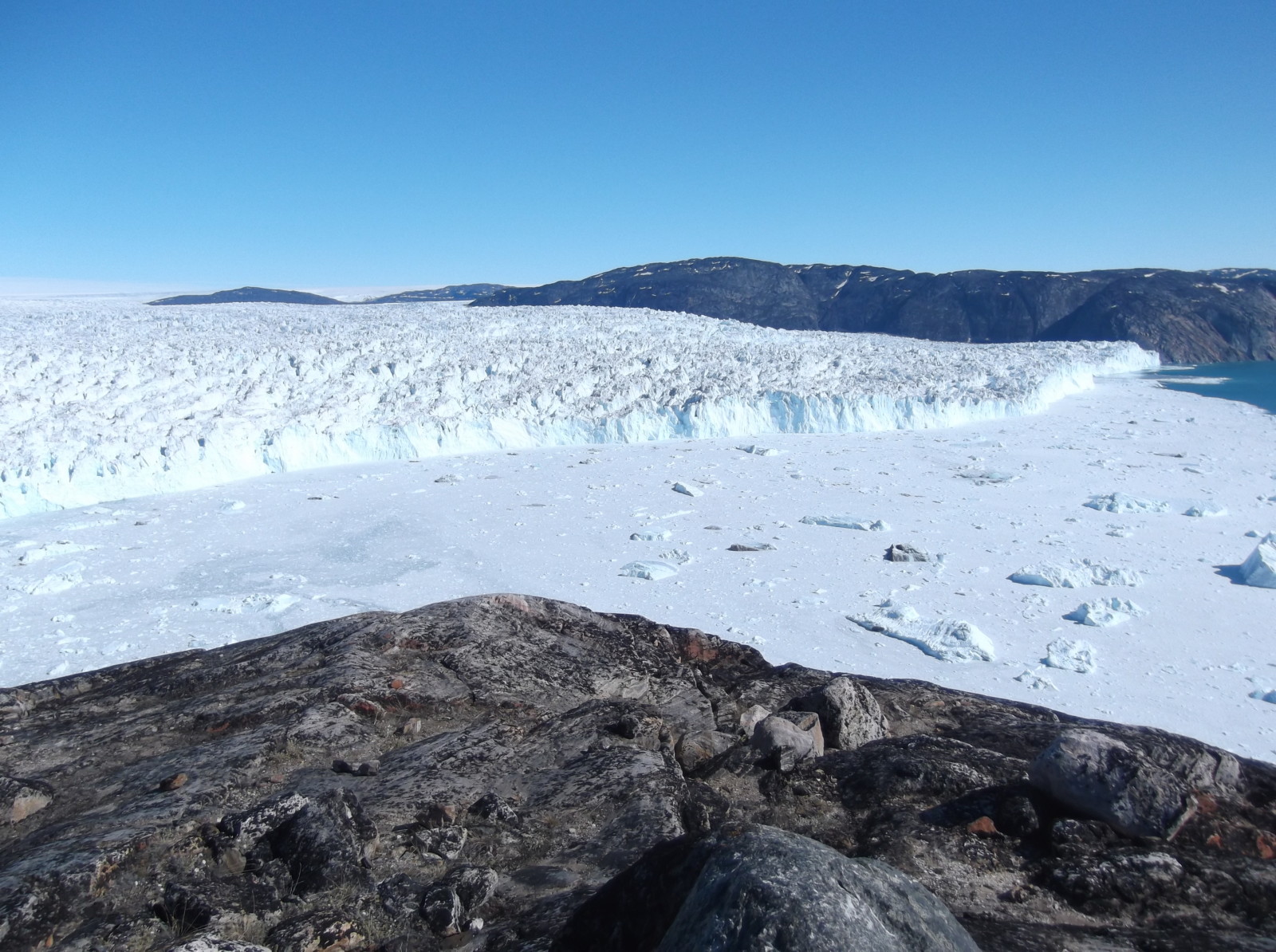
See what I mean about all the crevassing and sea ice near the front? Good luck getting anywhere near the outflow of any subglacial drainage.… Credit: Samuel Cook.
You can’t use radar to look at the subglacial drainage system either, because the resolution isn’t good enough and because the echoes tend to get confused at the base and by water content. You could use sonar, but you would run into the problem of not being able to get close enough in a boat, and you would also be at high risk of shipwreck from all the ice. So, you pretty much have to drill boreholes, but if you remember that near the terminus these glaciers are full of horrible crevasses, you will understand why this is not a great idea. One thing you can actually do is try to spot plumes of muddy meltwater by satellite – their sediment-laden water shows up well, and they make convenient holes in any sea-ice cover. But this still only tells you about the surfacing plumes – there will be many more that aren’t detectable from above.
You can see why people, such as me, generally try to model this on a computer. Not that that’s a panacea. The lack of observational data means that it is rather challenging to work out if the model output bears any resemblance to reality whatsoever. There are some fairly obvious sanity checks – if the model reckons the glacier is sitting on top of sheets of water that are tens of metres deep or channels that are kilometres wide, it’s probably wrong – but there an awful lot of different plausible solutions that it’s hard to discriminate between. There are ways of validating the model, don’t worry, but it’s surprisingly complicated for something that seems quite a straightforward step. There is hope, though – there’s a lot of work going on on using seismic data to track subglacial water flow, and new sensors have been designed to traverse the subglacial environment – so, with a bit of luck, people like me will have some more data to constrain our models soon enough! But, in an admittedly quite large nutshell, there’s an exposé of subglacial hydrology with a side order of why tidewater glaciers are evil.
Further reading
There’s a lot of possible reading out there on subglacial hydrology. I’ve suggested one or two articles (hopefully reasonably accessible – I’ve put review articles where possible) for each of the things I’ve covered, based on what I’m more familiar with, but these aren’t the only possibilities by a long shot.
Basics of subglacial hydrology
- Pick your favourite glaciological textbook – “Glaciers and Glaciation” by Benn and Evans (2010) is a good one.
Alpine-glacier hydrology
- Iken, A. and Bindschadler, R. A.: Combined measurements of Subglacial Water Pressure and Surface Velocity of Findelengletscher, Switzerland: Conclusions about Drainage System and Sliding Mechanism, Journal of Glaciology, 32(110), 101–119 (1986) DOI: 10.3189/S0022143000006936
- Mair, D., Willis, I., Fischer, U. H., Hubbard, B., Nienow, P. and Hubbard, A.: Hydrological controls on patterns of surface, internal and basal motion during three “‘spring events’”: Haut Glacier d’Arolla, Switzerland, Journal of Glaciology, 49(167), 555–567 (2003) DOI: 10.3189/172756503781830467
Greenland hydrology
- Davison, B. J., Sole, A. J., Livingstone, S. J., Cowton, T. R. and Nienow, P. W.: The influence of hydrology on the dynamics of land-terminating sectors of the Greenland Ice Sheet, Front. Earth Sci., 7 (2019) DOI: 10.3389/feart.2019.00010
- Nienow, P. W., Sole, A. J., Slater, D. A. and Cowton, T. R.: Recent Advances in Our Understanding of the Role of Meltwater in the Greenland Ice Sheet System, Curr Clim Change Rep, 3(4), 330–344 (2017) DOI: 10.1007/s40641-017-0083-9
Tidewater-glacier hydrology and plumes
- Hewitt, I. J.: Subglacial Plumes, Annual Reviews of Fluid Mechanics, 52, 145-169 (2019) DOI: 10.1146/annurev-fluid-010719-060252
- Slater, D. A., Nienow, P. W., Cowton, T. R., Goldberg, D. N. and Sole, A. J.: Effect of near-terminus subglacial hydrology on tidewater glacier submarine melt rates., Geophysical Research Letters, 42(8), 2861–2868 (2015) DOI: 10.1002/2014GL062494
- Sole, A. J., Mair, D. W. F., Nienow, P. W., Bartholomew, I. D., King, M. A., Burke, M. J. and Joughin, I.: Seasonal speedup of a Greenland marine-terminating outlet glacier forced by surface melt–induced changes in subglacial hydrology, Journal of Geophysical Research: Earth Surface, 116(F3) (2011) DOI: 10.1029/2010JF001948
Modelling of subglacial hydrology
- Cook, S. J., Christoffersen, P., Todd, J., Slater, D. and Chauché, N.: Coupled modelling of subglacial hydrology and calving-front melting at Store Glacier, West Greenland, The Cryosphere, 14(3), 905–924 (2020) DOI: 10.5194/tc-14-905-2020
- de Fleurian, B., Morlighem, M., Seroussi, H., Rignot, E., van den Broeke, M. R., Kuipers Munneke, P., Mouginot, J., Smeets, P. C. J. P. and Tedstone, A. J.: A modeling study of the effect of runoff variability on the effective pressure beneath Russell Glacier, West Greenland, J. Geophys. Res. Earth Surf., 121(10), (2016) DOI 10.1002/2016JF003842
Seismic investigation of subglacial hydrology
- Nanni, U., Gimbert, F., Vincent, C., Gräff, D., Walter, F., Piard, L. and Moreau, L.: Quantification of seasonal and diurnal dynamics of subglacial channels using seismic observations on an Alpine glacier, The Cryosphere, 14(5), 1475–1496 (2020) DOI: 10.5194/tc-14-1475-2020
- Vore, M. E., Bartholomaus, T. C., Winberry, J. P., Walter, J. I. and Amundson, J. M.: Seismic Tremor Reveals Spatial Organization and Temporal Changes of Subglacial Water System, Journal of Geophysical Research: Earth Surface, 124, 427-446 (2019) DOI: 10.1029/2018JF004819
Edited by Francesco Avanzi and Giovanni Baccolo
Samuel Cook is a postdoctoral researcher at Université Grenoble-Alpes. He recently completed a PhD at the University of Cambridge on numerical modelling of tidewater glaciers and is currently developing the use of data-assimilation methods in the Elmer/Ice numerical modelling suite as part of the MAGIC project.
Contact Email: samuel.cook@univ-grenoble-alpes.fr.