Recently, Machine Learning (ML) has emerged as a powerful tool within cryospheric sciences, offering innovative and effective solutions for observing, modelling and understanding the frozen regions of the Earth. From learning snowfall patterns and predicting avalanche dynamics to speeding up the process of modelling ice sheets, ML has transformed cryospheric sciences and bears many o ...[Read More]
Did you know? Machine learning can help us understand the cryosphere!
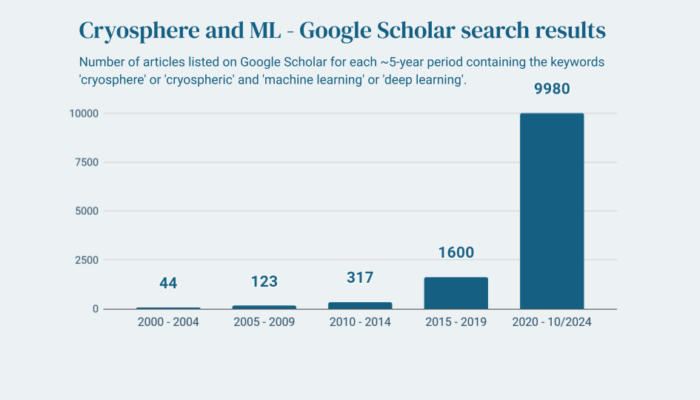