Particle Precipitation High energy particles (e.g. electrons and protons) that precipitate at high latitudes can alter the chemical composition of the atmosphere by different photochemical reactions. This mainly happens due to primary collision processes and subsequent ion and neutral-chemistry reactions. Such reactions ordered by increasing energy are, for example, excitation, photo-dissoc ...[Read More]
Exploring Magnetosheath Jets and their dependence on Solar Wind structures
Dayside of the Earth’s magnetic field Our understanding of the relationship between Earth’s magnetic field and the particles from the Sun that constantly bombard it, known as the solar wind, has significantly advanced in recent decades. The availability of numerous spacecraft measurements has provided valuable insights into this interaction. The solar wind, composed of high-speed particles c ...[Read More]
Why faculae “vanish” on active Suns
The Earth’s primary energy source is the radiative energy flux (i.e., the Total Solar Irradiance, TSI) from the Sun. Understanding the TSI or the solar irradiance output in particular wavelengths is important, as it is crucial for sustaining life of metabolisms on Earth and in answering the question of how life emerged on Earth. It has been shown that magnetic activity on the solar surface is one ...[Read More]
Employing J burst observations made by LOFAR to determine the properties of large coronal loops
Large coronal loops around one solar radius in altitude are an important connection between the solar wind and the low solar corona. However, their plasma properties are not well studied, as standard X-ray and UV techniques are not suited to these low-density environments. How does temperature, pressure, and magnetic field strength evolve along these loops? Observable structures in radio emission ...[Read More]
The impacts of space weather on the mid-latitude upper atmosphere
The upper atmosphere of Earth is constantly being impacted by the flow of charged particles being released from the sun. This flow (the solar wind) carries with it a magnetic field which distorts and reshapes that of Earth, ultimately resulting in a large amount of electromagnetic space weather energy being channelled into the polar regions. One of the most frequently observed outcomes of this pro ...[Read More]
Reflection after the investigation of the direct First Parker Solar Probe Observation of the Interaction of Two Successive ICMEs
In Heliophysics there is a scarcity of in-situ buoys that allows us to monitor and track the solar wind changes in our Sun’s atmosphere, the heliosphere. NASA’s Parker Solar Probe (PSP) mission is one of those buoys that, together with Solar Orbiter (SolO, ESA/NASA Collaboration), is bringing a breath of fresh air in the effort to fully characterize the solar wind and study the evolution of the em ...[Read More]
Initiation and Heliospheric Evolution of a stealthy solar eruption
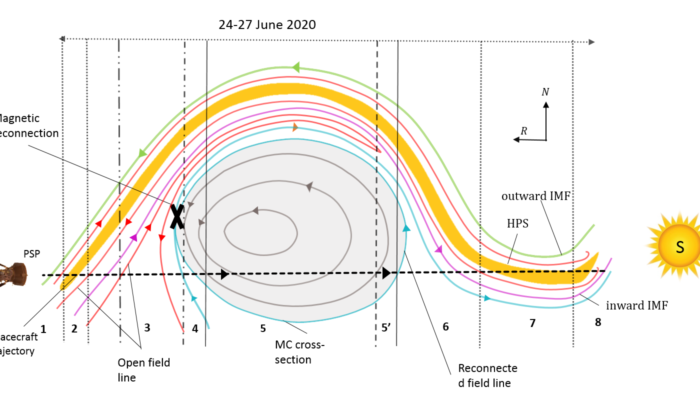
In the early 1970s, when Coronal mass ejections (CMEs), massive eruptions of magnetized solar plasma into interplanetary space, were first discovered, they were assumed to be always associated with the energetic solar phenomenon like solar flares, prominences, etc. With improved coronagraphic and multi-wavelength observational instruments, a class of CMEs emerging from the streamers was found and ...[Read More]
First year of Energetic Particle Measurements with EPD aboard Solar Orbiter
One year ago, the Energetic Particle Detector (EPD) aboard the European Space Agency’s (ESA’s) Sun observing spacecraft Solar Orbiter (SolO) was launched starting a long-awaited journey. SolO (Figure 1) will provide both in-situ and remote sensing measurements in the inner Heliosphere and EPD will contribute particularly to the latter ones. EPD consists of four sensors that share the s ...[Read More]
New auroral dunes discovered through citizen science
The region of the Earth’s atmosphere lying at altitudes between about 80 and 120 km, corresponding to the mesosphere–lower thermosphere–ionosphere (MLTI), is often referred to as the “ignorosphere”, because its observation is so challenging that only a handful of measurements of its composition, temperature and other physical parameters have been obtained over the last few decades. It is, however, ...[Read More]
Chasing solar storms as an early career scientist
Hello! My name is Erika Palmerio and I am a newly qualified Dr in space physics from the University of Helsinki, Finland. In this blog post I will talk about my PhD research and my future career plans. The title of my PhD dissertation is “Magnetic structure and geoeffectiveness of coronal mass ejections”. Coronal mass ejections (or CMEs) are huge and spectacular clouds of magnetic field and plasma ...[Read More]