The cryosphere comprises all the ice on Earth, from glaciers in Antarctica, icebergs floating in the ocean, to millimetric ice found in the soils. Soil can hold ice wedges and ice lenses, but there are small ice reservoirs that are usually overlooked: the pore ice. Pore ice is an unknown compartment whose role regarding matter degradation is yet to be determined… stay with us and you will see that ...[Read More]
UndercoverEisAgenten – Permafrost, drones and young explorers investigating Arctic change
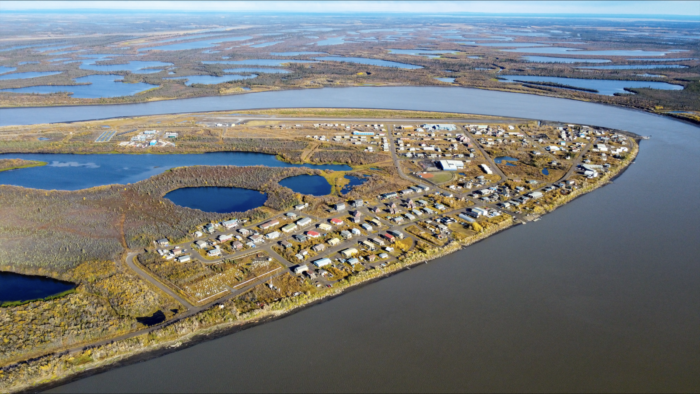
The “UndercoverEisAgenten” project takes us on a drone journey over the ever-changing permafrost landscapes. Initiated by the Alfred Wegener Institute (AWI) for Polar and Marine Research, the German Aerospace Center’s (DLR) Institute of Data Science, and the Heidelberg Institute for Geoinformation Technology (HeiGIT), this venture is about more than just capturing images. It̵ ...[Read More]
Did you know… about worms surviving in permafrost for at least 46000 years?
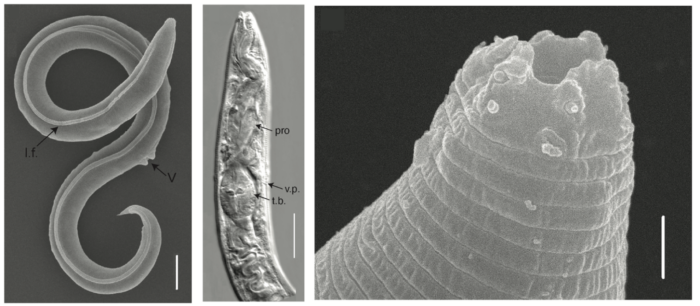
Lately permafrost makes the news more and more because of its enormous carbon stocks and its vulnerability to climate change. While permafrost greenhouse gas budget calculations are complex and harbour an ever-growing research community, its microbial ecology is still on the rise. A recent star are tiny roundworms that survived frozen in permafrost for 46’000 years. Take a short dip into this new ...[Read More]
Small step for reindeer – large leap for humankind?
Since several decades, there’s a lot of discussion in the permafrost ecosystem community on “rewilding” and “return to a natural state” in order to protect ecosystems and to reduce the impacts of climate change. Reindeer and other herbivores influence the insulation regime of the ground and could thereby preserve the frozen state of permafrost ground. Is there a way to utilise this effect to our b ...[Read More]
Fostering connections with frozen landscapes
Whether they are natural occurrences or experimentally induced, permafrost and Arctic ecosystems are responding fundamentally to recent climate extremes. Good writing and statistics material for us scientists, but bad news on pretty much every other level. Are we effectively communicating how fundamental these changes are? And can we use our personal experiences, stories and artwork to support us ...[Read More]
Perspective on Listening to Permafrost
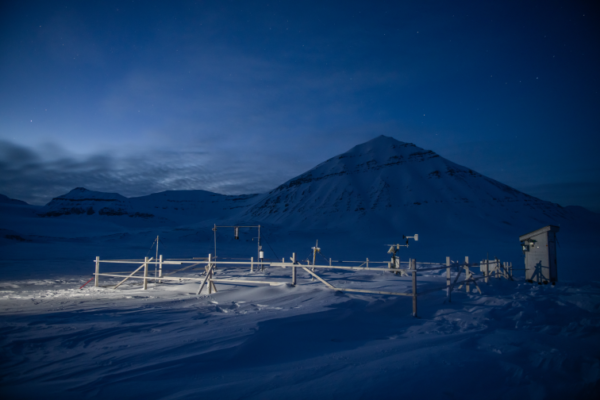
> Reflections from an ongoing art-science research project Common Grounds < How to visualize thousands of thousands (> 20,000,000) of data points collected in the Arctic atmosphere and subsurface at a permafrost observatory over 25 years? How to tell the story of permafrost, ground that is permanently frozen and an important part of the cryosphere, that is currently warming and thawing at ...[Read More]
Did you know that thawing permafrost is impacting Arctic livelihoods already today?
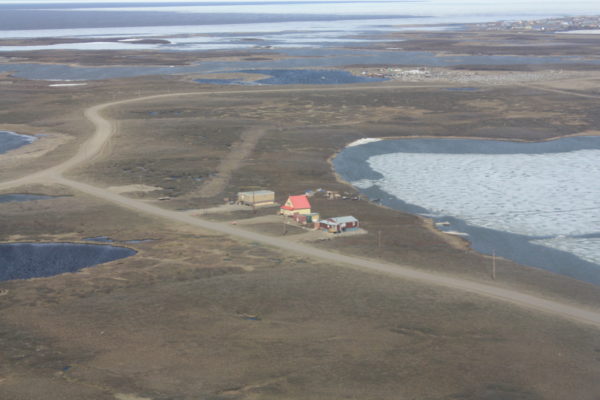
Would you like to join me on a little trip up North today? We will be visiting a small community in the Canadian high Arctic – a community built on permafrost ground. This sounds pretty cool in the first place, but brings along quite a few challenges that most of us probably do not have to think about. Let me introduce you to Tuktoyaktuk, the community that is endangered to fall into the sea ...[Read More]
End-of-the-year special: this year’s Cryoblog
So this is the last post in 2022 for our blog. We have decided that this time, the topic will not be another exciting story about the science of ice and cold in their various forms. This time we are talking about the blog itself, so a kind of meta-post to take stock and understand a little better how our blog works, what it is about, and who our main authors are. To this purpose, we asked all the ...[Read More]
Wake up every one – permafrost microbes feasting – greenhouse gases go
I really like to combine science and art. This puts science in a larger perspective and can help understand it in different ways. And perhaps more importantly, it evokes emotion. I wrote haikus and created stencils for every chapter of my PhD thesis. I created these artworks to illustrate the dimensions of the permafrost region and the consequences of global warming on permafrost. Let me show you ...[Read More]
The intriguing order of cold terrains
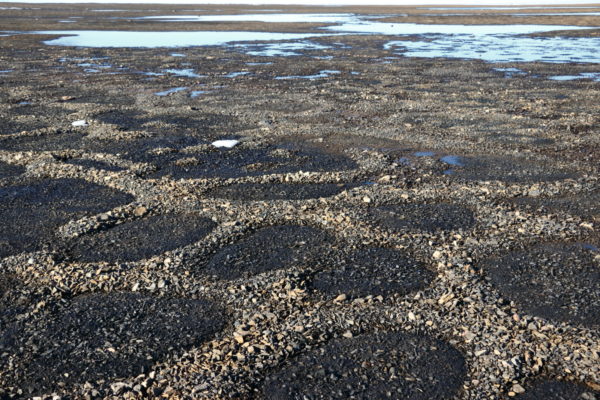
Do you know what the periglacial environment is? Well, the word periglacial refers to those environments which are somehow sculpted by seasonal freeze and thaw cycles. The alternation of freezing and thawing conditions can change the landscape, creating some spectacular landforms. Stone circles are certainly among the most mysterious and fascinating. Come and discover them! Some definitions Glacia ...[Read More]