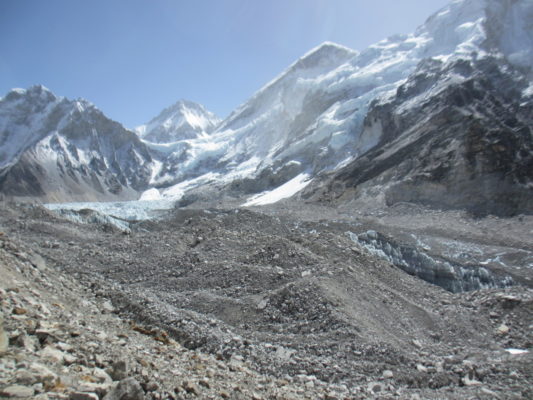
We know that glaciers are actively responding to climate change, but what is happening on the inside? The conditions within a glacier strongly influence its behaviour, but the deep and dark depths of a glacier are difficult to access – we know very little about this remote environment. The EverDrill project (2016 – 2019), funded by the UK Natural Environment Research Council, aimed to fill this knowledge gap by drilling into a debris-covered glacier and collecting unprecedented data from its interior. Now that the field seasons have been completed and most of the data analysed, what light have we shed on the dark interior of this debris-covered mountain glacier?
Mountain Glaciers
The increasing loss of ice due to climate change and associated implications, particularly for mountain glaciers, is a “hot” topic at the moment (Hock et al., 2019). In order to forecast how long glaciers might last in different warming scenarios, scientists use numerical models, fed and validated by data from the glaciers themselves. However, in mountain environments, such data are limited due to the difficulty in accessing these glaciers. This is particularly the case for debris-covered glaciers, whose surface is covered by a layer of rocks and sediment (Figure 1), making it even harder to take any measurements from the glacier itself.
Drilling
To try and fill a part of this data void, the EverDrill project aimed to access the interior of Khumbu Glacier, Nepal – perhaps one of the best-known debris-covered glaciers due to its infamous icefall that provides a route for climbers aiming to summit Mt. Everest. One way to access the interior of a glacier is to bore a hole from the surface through the ice. Such boreholes were melted into the glacier using a hot-water drill, each reaching between 1 – 192 m in depth. Over two field seasons, 27 boreholes at five sites across the glacier’s ablation area (the part of the glacier where mass is lost, rather than gained) were drilled into Khumbu Glacier, with a cumulative 760 m of borehole (Miles et al., 2019). The longest boreholes at each site were logged and instrumented with probes to measure different aspects of the glacier’s interior.
Ice Temperature
The temperature of the glacier ice was measured at multiple depths in each instrumented borehole by strings of temperature probes, for up to two years. The results showed much warmer ice temperatures than we expected to find (Figure 3). The coldest ice temperature measured was –3.3°C, far warmer than the mean annual air temperature (MAAT) of –9 to –13°C where the ice forms on the southern flanks of Mt. Everest. Comparison with one measurement made farther upglacier in 1974 suggests that the ice may have warmed by ~2–3°C in the past 43 years, and at all sites, the ice temperatures were warmer (by up to 2°C) than the MAAT for that elevation (Miles et al., 2018).
As a whole, these results demonstrate that over half of Khumbu Glacier’s ablation area contains “temperate” ice – defined by glaciologists as ice that is at the melting-point temperature. When additional heat is applied to temperate ice, the ice melts to become water. By comparison, “cold” ice (ice colder in temperature than the melting-point) simply becomes less cold when more heat is applied. Khumbu Glacier may therefore be more sensitive to future increases in temperature than previously thought, particularly as increasing air temperatures are amplified in high mountain regions (Pepin et al., 2015). As glaciers feed some of the largest river catchments across the world, this could have implications for future water resources (Immerzeel et al., 2020).
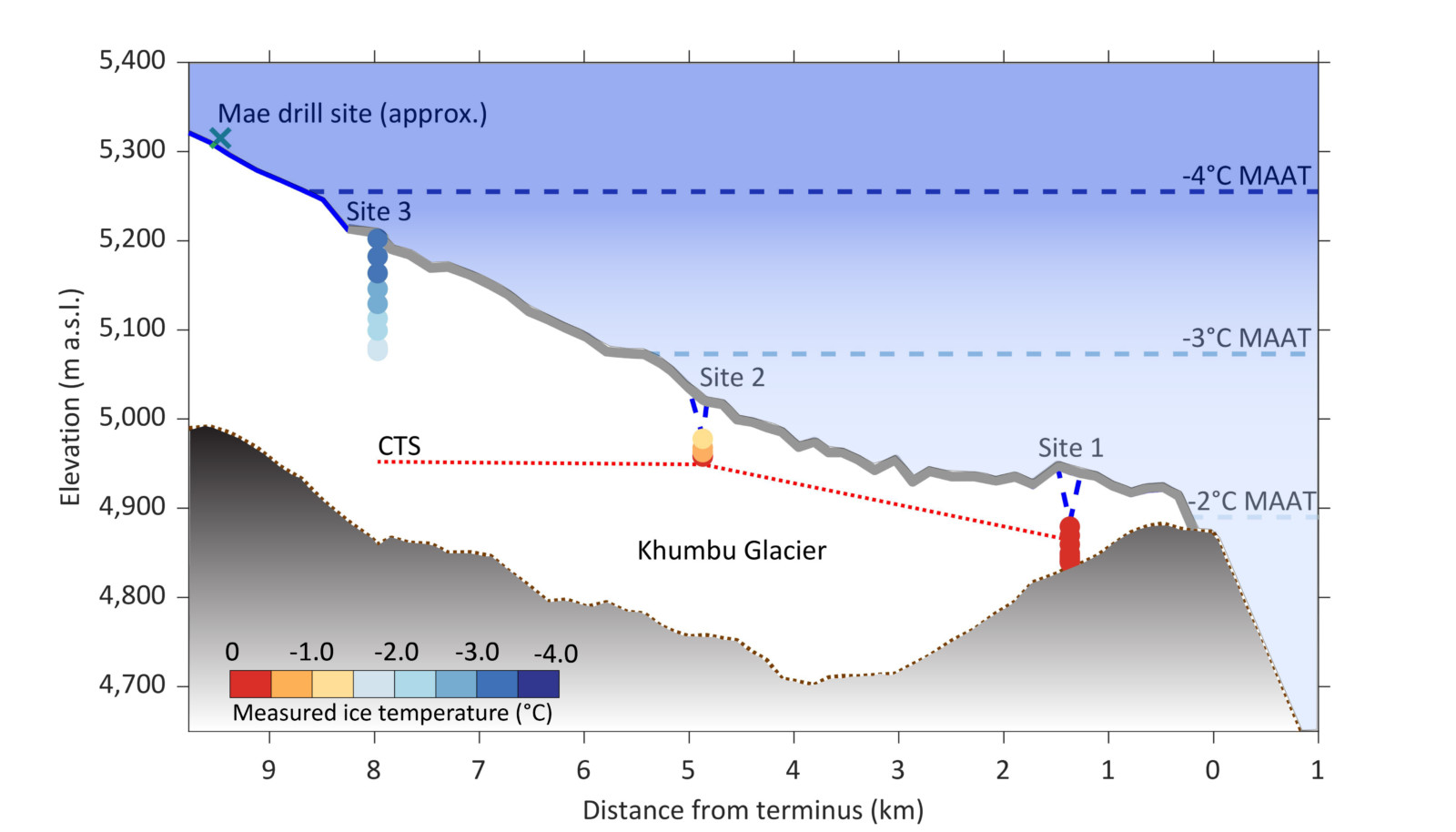
Figure 3 – Long profile of Khumbu Glacier showing ice temperatures recorded by each thermistor in three boreholes, from Miles et al. (2018). The cold-temperate transition surface (CTS) indicates the estimated division between “temperate” and “cold” ice (below and above the dotted line, respectively). Mean annual air temperatures (MAAT) are calculated from the 1994 – 2013 valley lapse rate (Salerno et al., 2015).
Debris Content
However, it is not all doom and gloom – particularly for debris-covered glaciers. While thin surface debris layers enhance ice melt, where the surface debris layer is more than a few centimetres thick it acts as a blanket that insulates the ice from short-term changes in air temperature (Østrem, 1959). This effect may – to some extent – shield debris-covered glaciers from warming air temperatures, particularly as debris-layer extents are increasing worldwide. Debris layers are primarily created from rock-laden avalanches onto the glacier surface, and in the upper reaches of the glacier, this can result in debris being buried with the snow and becoming entrained with the glacier ice. Downglacier, this internal debris will later melt out with the ice, adding to the thickness of the surface debris layer and potentially protecting the glacier from future air temperature increases.
We estimated the amount of englacial debris in each of our boreholes from image logs taken using an optical televiewer – a 360°, high-resolution borehole camera. In the image logs, we could see debris ranging in size from fine sediment to small clasts sticking out from the borehole wall (Figure 4). Our estimates showed an increase in the englacial debris content from the upper ablation area (~0.7% by volume) to the lower ablation area (~6.4% by volume) – with higher mean values than have been observed in the small number of studies conducted elsewhere – and an increase in englacial debris content with depth (Miles et al., 2021). While this would appear to provide plenty of debris to insulate the glacier surface, this englacial debris has to get to the surface first, involving the melt of a lot of ice – in the upper ablation area, our 150 m borehole would produce a debris thickness of ~1.5 m if all the ice were to melt.
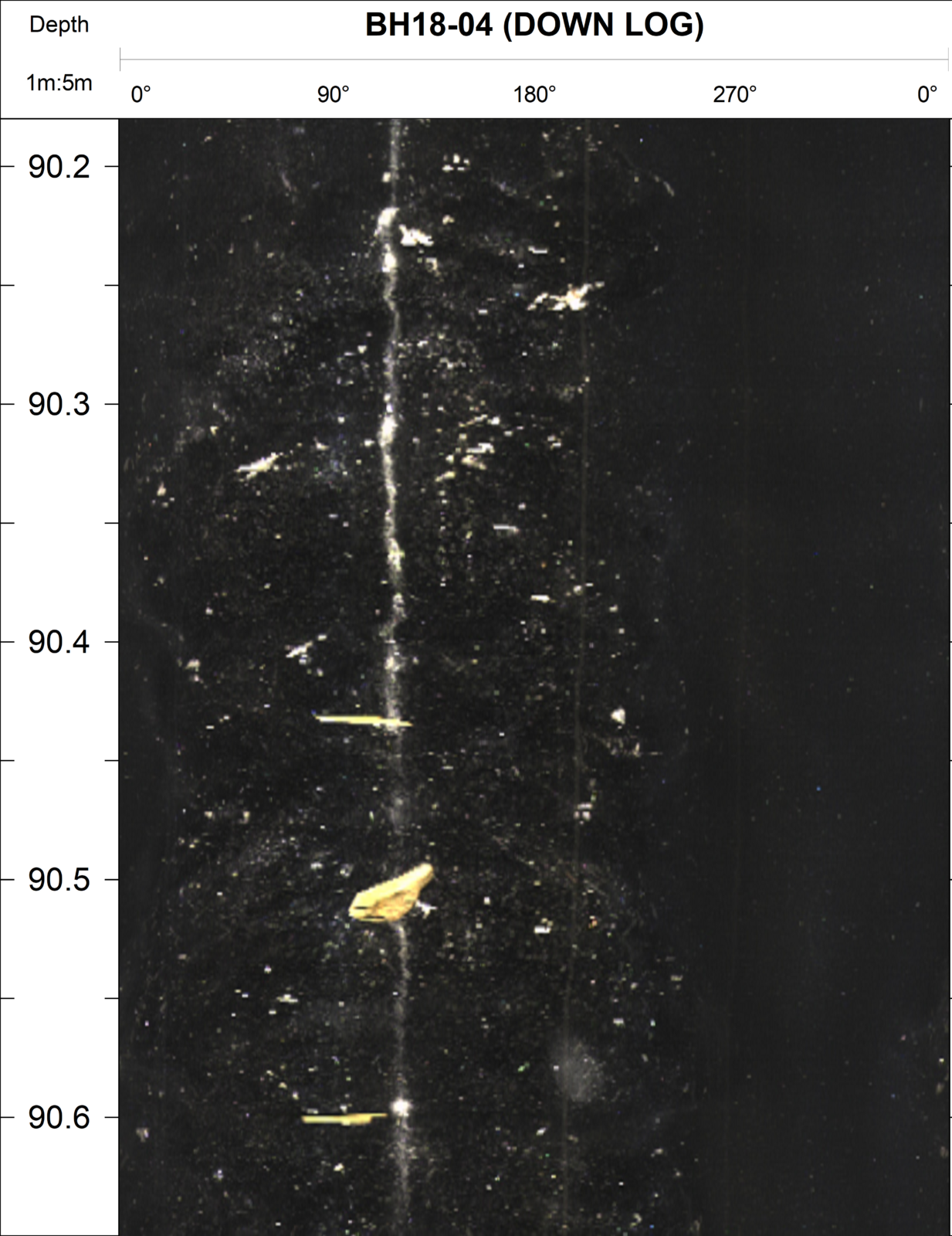
Figure 4 – An unrolled section of an OPTV image log, showing fine debris and larger debris clasts (red-gold hues). The borehole circumference (image width) is ~30 cm. Credit: the author.
What next?
Now we have collected and analysed the data, what next? With our new and improved understanding of both the ice temperature and distribution of debris within Khumbu Glacier, the next step for the EverDrill project is to constrain the influence of these properties on the glacier’s lifespan. To do this, we will include our data in a numerical model of the glacier (Rowan et al., 2015) and compare the results to previous simulations without such data. Detailed variations in ice temperature and englacial debris with depth are currently not included, so the contrasting impact of these two processes will be interesting to investigate. Watch this space!
Further Readings:
- Hock et al. (2019) “High Mountain Areas” in IPCC Special Report on the Ocean and Cryosphere in a Changing Climate
- Immerzeel et al. (2020) “Importance and vulnerability of the world’s water towers” Nature 577:364–369, DOI: 10.1038/s41586-019-1822-y.
- Miles et al. (2021) “Continuous borehole optical televiewing reveals variable englacial debris concentrations at Khumbu Glacier, Nepal” Commun. Earth Environ. 2:1–9, DOI: 10.1038/s43247-020-00070-x.
- Miles et al. (2018) “Polythermal structure of a Himalayan debris-covered glacier revealed by borehole thermometry” Sci. Rep. 8:1–9, DOI: 10.1038/s41598-018-34327-5.
- Miles et al. (2019) “Instruments and methods: hot-water borehole drilling at a high-elevation debris-covered glacier” J. Glaciol. 65:882–832, DOI: 10.1017/jog.2019.49.
- Østrem (1959) “Ice Melting under a Thin Layer of Moraine, and the Existence of Ice Cores in Moraine Ridges” Geogr. Ann. 41:228–230, DOI: 10.1080/20014422.1959.11907953.
- Pepin et al. (2015) “Elevation-dependent warming in mountain regions of the world” Nat. Clim. Chang. 5:424–430, DOI: 10.1038/nclimate2563.
- Rowan et al. (2015) “Modelling the feedbacks between mass balance, ice flow and debris transport to predict the response to climate change of debris-covered glaciers in the Himalaya” Earth Planet. Sci. Lett. 430:427–438, DOI: 10.1016/j.epsl.2015.09.004.
- Salerno et al. (2015) “Weak precipitation, warm winters and springs impact glaciers of south slopes of Mt. Everest (central Himalaya) in the last 2 decades (1994-2013)” Cryosph. 9:1229–1247, DOI: 10.5194/tc-9-1229-2015.
Edited by Giovanni Baccolo
Katie Miles is an Associate Lecturer at Aberystwyth University, UK, where she also obtained her PhD. Her research involves field-based investigations of the internal structure and subsurface hydrology of ice masses. She tweets at @Katie_Miles_851 and can be emailed at kam64@aber.ac.uk.