Summer time can be stressful – you have plenty of things to do at work but at the same time you’re thinking about your next vacation all the time. This is at least how I was feeling, combined with some curiosity, I asked chatGPT to write a blog post about vacation destinations for geologist around volcanoes in Europe – here are the results, I hope you get inspired: Calling all g ...[Read More]
Volcanoes and Corals: a journey to discover volcanic activity as seen from a coral perspective with Prof. Tom Sheldrake
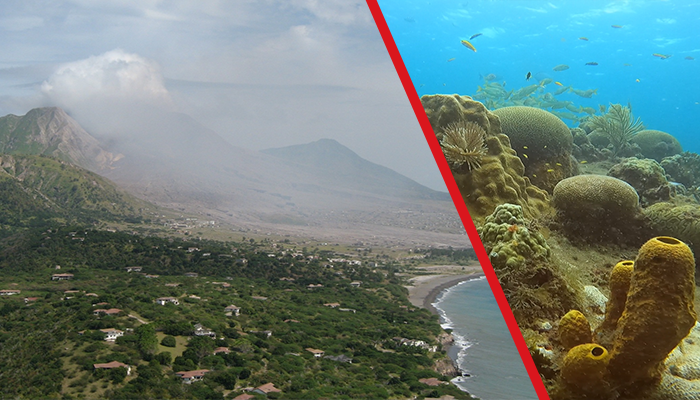
What can corals tell us about volcanic eruptions? Is it possible corals, in the span of their lives, have recorded valuable data on volcanic eruptions they have witnessed? Today we find it out together with Prof. Tom Sheldrake, who takes us on a journey through coral reefs and volcanic eruptions, giving voice to what corals want to tell us about volcanic activity! Introducing Professor Tom Sheldra ...[Read More]
I asked ChatGPT to write a blog about lava lakes: here’s what happened
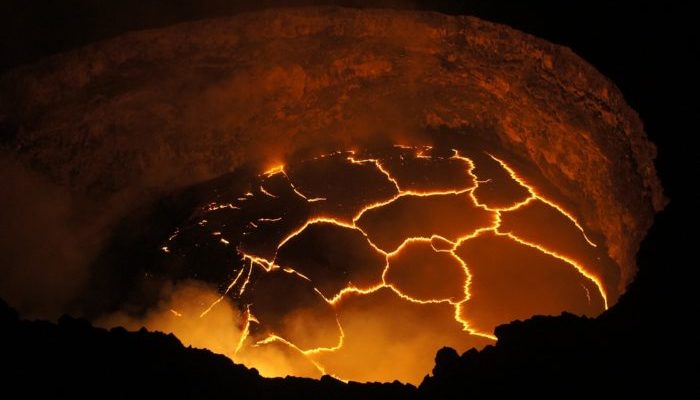
If you’ve not been living under a rock you’ve probably heard of ChatGPT, the AI text generator wowing the internet and striking fear into the heart of exam co-ordinators worldwide. I’ve been meaning to write a blog about lava lakes for a while now, ever since rewatching Werner Herzog and Clive Oppenheimer’s excellent documentary Into the Inferno. But with deadlines looming and lab work and admin c ...[Read More]
Geo-Fantasy – between Fantasy Novel and the Real World
When reading fantasy novels, we are usually brought to worlds of elves, dragons, and epic battles, all surrounded by breathtaking magical forests, or castles above impervious cliffs. However, even fantasy stories take inspiration from the real world and its geology. Who does not remember the ascent of Mount Doom of Frodo and Sam, or the importance of Dragon Glass (Obsidian) in Game of Thrones? So, ...[Read More]