There is still a huge amount we don’t know about how glaciers respond to climate change. One of the most challenging areas is determining the response of debris-covered glaciers. Previously, we have reported on a number of fieldwork expeditions to debris-covered glaciers but with this Image of The Week we want to show you another way to investigate these complex glaciers – numerical modelling! Deb ...[Read More]
Image of the Week – Supraglacial debris variations in space and time!
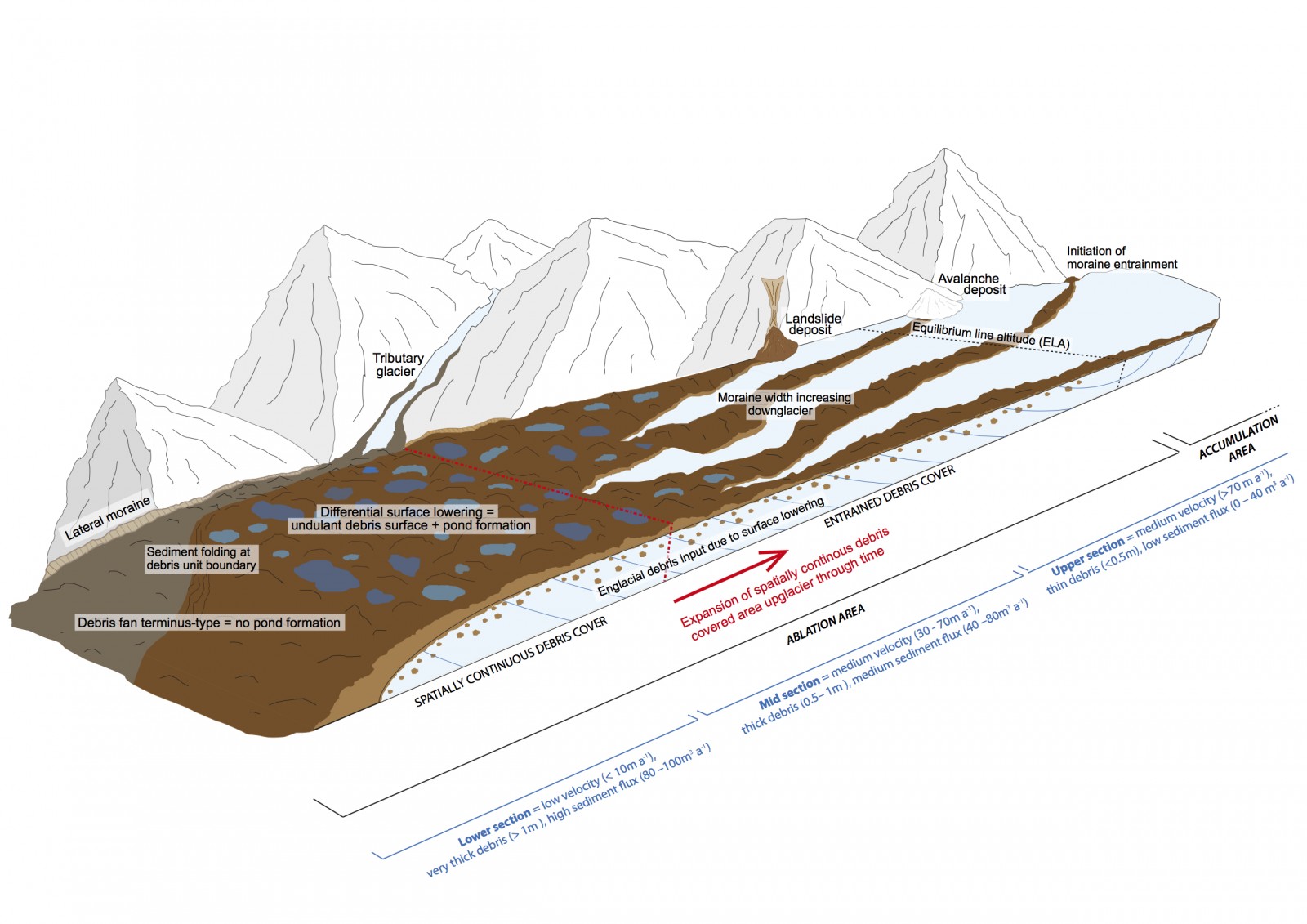