– A debate among scientists and its impact on us The epoch of humans (and their obvious intervention in the Earth system) In order to understand what the ‘Anthropocene’ means for us, we need to define first what it actually is. This poses a rather complex question in itself, as various disciplines have given the term rather different and alternative definitions. For instance, the public medi ...[Read More]
EGU Climate Division presents: Outreach Team 2023 edition
The European Geosciences Union (EGU) is a multidisciplinary organization, encompassing various fields within the geosciences. Each field is represented by its own Division, within which a number of volunteer roles exist. These roles include the President and Deputy President, a Programme Group Chair, Science Officers, Early Career Scientist Representatives, and an Outreach Team. Every year at the ...[Read More]
Past climate of the Central North Atlantic, insights from ancient soils in the Azores volcanic islands
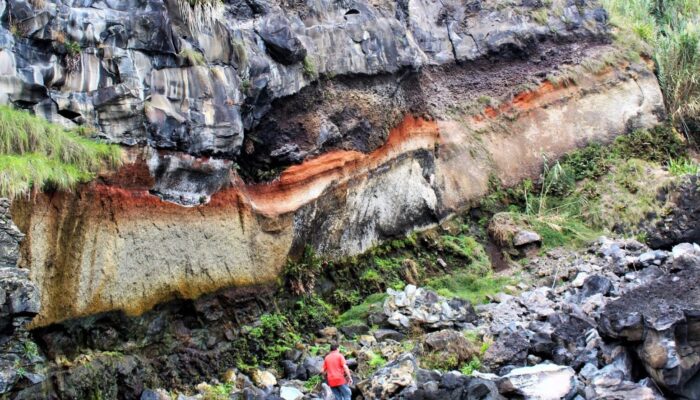
The Atlantic Ocean, a climate driver for Europe and North America The climate of both Europe and North America is influenced by the conditions of the Atlantic Ocean. This teleconnection between the ocean and the atmosphere has been the focus of great interest, especially in the context of ongoing global warming. For instance, Cresswell-Clay et al. (2022) noted an expansion of the North Atlantic Os ...[Read More]
Put a Climate lab in school and make it better!
Put a Climate lab in school and make it better “The car will not go through this road”, the driver replied. Well, the path, which he was mentioning was a mudslide made up of clay soil, completely wet from the rain the previous day. We were traveling to a small village in Sundarban to establish a climate laboratory. The concrete road ends 2 kilometers before the schools, and then it was a mud bath! ...[Read More]