The EGU Geochemistry, Mineralogy, Petrology and Volcanology ECS committee is currently looking for early career scientists to present at and attend our monthly online talk series (EGU Campfires). The EGU Campfires are a free one-hour online seminar consisting of three 10-12-minute talks. These quick-fire presentations give you an opportunity to share your work in a relaxed and friendly environmen ...[Read More]
How can fossil Geothermal System enhance Present Exploration?
Geothermal resource development faces a major obstacle: the expensive exploration process. Unsuccessful drilled wells can discourage interest and disrupt economic plans. Understanding the relationship between geologic structures and geothermal fluid flow is critical to successful exploration. Various methods, such as geochemistry, geophysics, structural analysis and modeling, aim to create a relia ...[Read More]
The role of Geothermal Energy in the energetic and environmental challenge.
How does a Geothermal system work? Heat is a form of energy and, strictly speaking, geothermal energy is heat from inside the Earth. The large amount of thermal energy enclosed below the earth’s surface derives in part from its primordial formation process and in part originates from the decay of radioactive isotopes present mainly in the earth’s crust and, secondarily, in the mantle. ...[Read More]
Paper focus: Plumbing the depths of magma crystallization by Hugo Moreira and co-authors
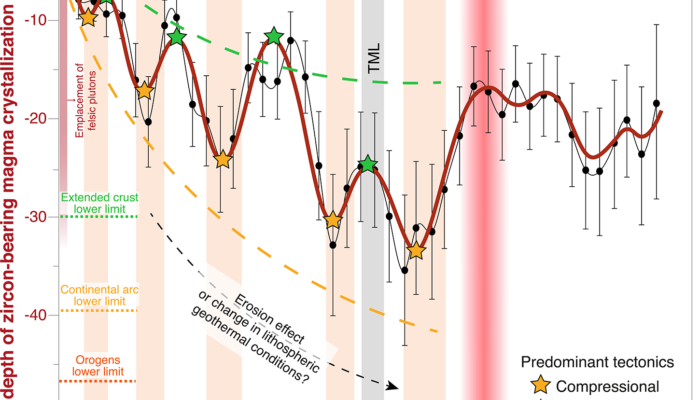
Do you know when you stumble upon a very interesting paper, with a captivating title and you wish you could talk to the author(s)? Well, I did just that. It happens that I know the first author quite well, so I had no shame in contacting him and ask about it. One of his latest papers was published in Geology with the title “Plumbing the depths of magma crystallization using 176Lu/177Hf in zi ...[Read More]