We all know that cement is a man-made substance and therefore cement is always synthetic right? Wrong!
In the unusual case of Maqarin, Jordan the stars aligned to produce natural cement and many of the “synthetic” minerals found therein. The Maqarin site has been the subject of an intense geological investigation by a consortium of over 100 researchers for years in an attempt to try and understand this system and its implications. A fairly good overview is available here. Maqarin is located in north-east Jordan, near the border with Syria, in the river valley of the Yarmouk River. The valley is deeply incised allowing a good view of the stratigraphy. There are also several springs coming out of the sides of the valley wall and it is these and their relationship to the unusual geology that make Maqarin so interesting.

A map of Jordan. The location of Maqarin is starred. (Source)
Geologic History
The geologic history of the Maqarin site is very interesting and in many ways unique. Indeed, it was a unique combination of geology and geological events that led to its formation.
1. During the Cretaceous bituminous marls are formed. Basically, rocks that are full of bitumen and calcium carbonate. This rock has up to 20% organic matter! Bitumen forms when organic matter is heated during lithification and is similar to oil. It will burn. The marl is a white, chalky mineral primarily made of CaCO3. There is also about 10% sulphate, which becomes important later on in the geologic history.
2. Overlying the bituminous marls are chalky limestones that were deposited in the Tertiary.
3. The upper unit is basalt, which was deposited during the Pleistocene.
4. The bituminous marl underwent “pyro-metamorphism”. Basically, the rocks were heated to a point where the carbonate rocks cooked and formed high temperature cement minerals such as portlandite, ettringite and thaumasite. The heating though was not due to burial but actually from spontaneous combustion of the bitumen (Khoury et. al., 1992). The presence of sulphate allowed these minerals to form and better simulated a modern cement environment. When the marl, which is CaCO3, is combusted CaO or lime is formed. Then the infiltrating groundwater allows portlandite to form, which then reacted with the suplhates and silicates present to form the other cement minerals.
5. The rocks in the area are all highly fractured allowing water to easily seep through the site. This has led to weathering of the cements. The water seeping through the sites discharges near by and has a pH of 13!!!! The water that seeps through the “cement zone” of the Maqarin site picks up all sorts of dissolved ions and minerals. Remember that heating carbonate + water gives Ca(OH)2. Therefore, there is a huge supply of hydroxide available to raise the pH.
These 5 steps created the Maqarin analogue site as we see it now. Everything had to be perfect for it’s formation to happen: the rocks had to have the right chemistry, pyro-metamorphism had to occur and, the hydrogeology had to allow water to infiltrate and react with the rocks to produce the hyperalkaline springs. See the references below for a more detailed description of the geology and mineralogy of Maqarin.

An image of the Yarmouk River Valley from the Jordan Times. Notice the bedded cliffs along the river. At Maqarin it is these cliffs that have the springs in them. In the distace you can also make out the sraker, overlying basalt. (Source)
Some Geochemistry
I mentioned the “hyper-alkaline springs” above. These springs are super interesting to geochemists, since there not very many places on Earth where we can find springs of highly elevated pH. Indeed, it is much more common to find acidic springs than alkaline ones. However, the springs of Maqarin have exceptionally high pH’s. Why?
The alkaline springs, which flow out of the hillsides at Maqarin, formed due to the unique combination of the hydrogeology, structural geology and mineralogy of the site. As the water infiltrates through fractures in the rock it intially dissolves NaOH and KOH followed by Ca(OH)2, which is the cement mineral portlandite. All of the OH ions that enter solution contribute to the raising of the pH to around 12.5. This now caustic water is then able to react with other minerals and pick up a lot of metals such as uranium, chromium, vanadium and other exotic elements that would usually be insoluble and hence difficult to transport (Khoury et. al, 1992).
The stable isotopes also tell and interesting story about the origins of the water and its pathways at Maqarin. In arid climates, such as Jordan, the groundwater often reflects evaporation that took place either before or during recharge. The waters of Maqarin plot below the local meteoric water line, which is indeed indicative of evaporation before or during recharge of the groundwater. However, there is more to these waters than just evaporation. In fact, the hydration of the cement minerals, such as portlandite, can cause an 18O enrichment, which can also be seen in the Maqarin groundwaters, although it is difficult to differentiate this effect from that of evaporation, which also produces the same trend (Khoury et. al. 1992).

Plot of 18O vs 2H in the hyperalkaline springs of Maqarin, Jordan. The local meteoric water line (Irbid) and the eastern Mediterranean meteoric water line are both shown. Modified from Khoury et. al., 1992.
Why study Maqarin?
We need to understand how cement will behave over long time scales because in most nuclear waste storage or carbon sequestration designs one of the primary barriers between radionuclides/CO2 and the environment are the engineered barriers, many of which are made of cement. In fact, in a repository setting cement is everywhere. It will be used to contain the waste itself, plug up fractures, reinforce structures, and eventually to seal the whole place up. Sealing the place up water-tight (hopefully radio-nuclide tight also) is great, but what happens to the seals when they are subjected to a million years of groundwater flow? Well, the modelling suggests that…. Wait, did I just say modelling? You mean we rely on computer generated geochemical models to tell us what might happen in a number of scenarios? We don’t know exactly what will happen to all that cement that is plugging up a waste site?
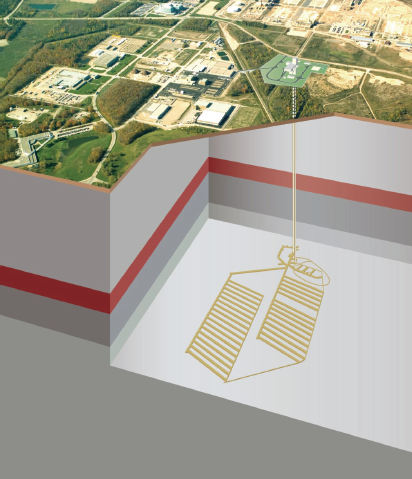
An artists depiction of a deep geologic repository for low and intermediate level radioactive waste. (Source)
Enter Maqarin. Maqarin is such an interesting place because “cement” occurs naturally here and it provides a rare opportunity for researchers to actually see how cement and the minerals composing it behave over long time scales when subjected to weathering processes. Therefore, the site can be considered an analogue for the future behaviour of cement in the environment. Indeed, the hydration process that occurs in Maqarin when the groundwater reacts with NaOH, KOH and Ca(OH)2 is exactly what will happen when “man-made” cement is exposed to groundwater. Understanding the geochemistry and hydrogeology of a site like Maqarin gives real-world insights into the outcome of our modelling and we can take the data collected at places like Maqarin and compare them to our model results to verify its accuracy. Furthermore, using observations and empirical data to make conclusions about the future of waste repositories adds a whole new level of confidence in their design or identifies possible weaknesses.
In summary, since radioactive waste storage facilities, which are designed to have million year lifespans, are made of cement it is crucial that we know what will happen to them over long time periods. However, the problem is that modern cement is just that: modern. Therefore, we don’t really know how it will behave in certain environments or when conditions change, or during an ice age, etc, etc. In order to solve this problem we must turn to the next best thing, which is a natural site, such as Maqarin, that mimics the environmental conditions we are looking for and hopefully it can answer some of these questions. Hence, the beauty of the natural analogue.
Thanks for reading,
Matt
References
Khoury, H.N., Salameh, E., Abdul-Jaber, Q., 1985. Characteristics of an Unusual Highly Alkaline Water from the Maqarin Area, Northern Jordan. Journal of Hydrology 81, 79–91.
Khoury, H.N., Salameh, E., Clark, I.D., Fritz, P., Bajjali, W., Milodowski, a. E., Cave, M.R., Alexander, W.R., 1992. A natural analogue of high pH cement pore waters from the Maqarin area of northern Jordan. I: introduction to the site. Journal of Geochemical Exploration 46, 117–132.