Sea ice is an interesting phenomenon, especially to a Canadian. The question around this time of year that always arises in the news is will this be a big sea ice year, will we set a new record low, high (haha) or will it be just average? This is a question that gets a lot of study and media attention. People run countless statistical models to predict sea ice conditions and try to predict the past and future conditions based on these annual observations. It is a nerdy admission but there are two things I follow religiously on the web: sea ice coverage and NHL standings (Go Leafs!!)….ah, who I am kidding, it is mostly about sea ice. So, you can imagine my interest the other day when I came across an article on the CBC about a new proxy for measuring past sea ice coverage. Even stranger was that fact that this new proxy was corralline algae, which I had been looking at in thin section earlier that day in my role as a carbonate sedimentology TA. It is a strange ol’ world.
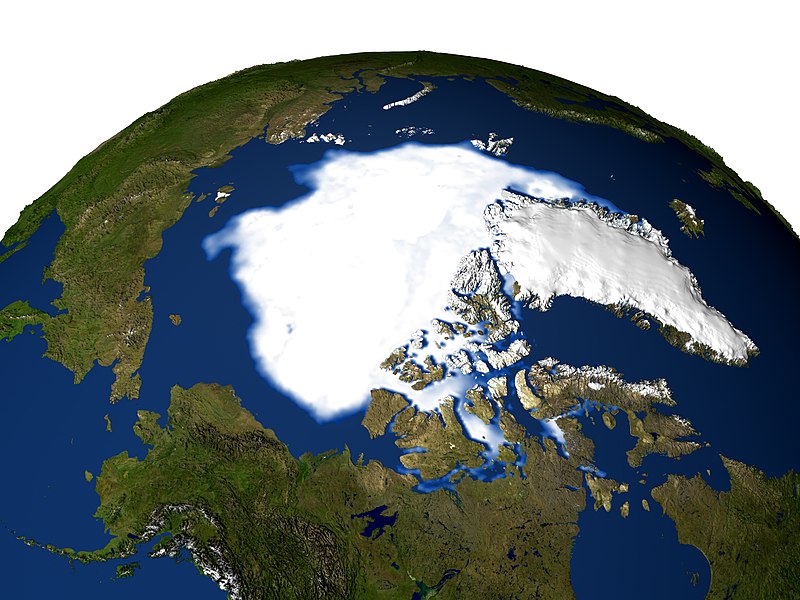
2005 NASA Sea Ice extent Imagery. (Source) – Wikimedia Commons – NASA
Anyway, this new paper entitled “Arctic sea-ice decline archived by multicentury annual resolution record from crustose corraline algal proxy” recently appeared in PNAS. In this study the authors used corralline algae as a new proxy to estimate sea ice coverage in the Arctic. The question that these authors sought to answer using corraline algae as a proxy was: can we calculate past sea ice coverage and can we then use the larger knowledge base we now have about past sea ice to apply that to predicting future sea ice conditions given at current models are not very reliable at predicting future sea ice coverage?
First, lets look at this corraline algae proxy. Corraline algae is a type of red algae that have been around since Cretaceous. As the name suggests they tend to be red in colour and often grow in coral reefs. According to Wikipedia appear as “pink to pinkish-grey patches, splashed as though by a mad painter over rock surfaces”. (How poetic eh?). Anyway, they are widely distributed globally and occur in all the world’s oceans up to the maximum depth light can penetrate as they are photosynthetic and their growth depends on light and temperature. The species of corraline algae used in this study was Clathromorphum compactum, which forms a new layer ever year, much like a tree forms rings. Clathromorphum is an encrusting variety of red algae that grows on rocky substrates year after year essentailly in perpetuity as the only thing that can prevent it from adding another high Mg calcite later is if something actually breaks it apart or eats it. The authors found samples of Clathromorphum up to 646 years old!

Photo of coralline algae – Wikimedia Commons – Author: Derek Keats
The way a proxy works is that a certain feature of the sample, such as its chemistry or growth ring width, can be used to tell us about conditions that were responsible for chemical changes in the sample or the size of its growth rings. It is even better if the sample can be used to represent large time periods. Therefore a sample of 646 year corralline algae has the potential to be used as a proxy if its chemical or growth features are caused by a change in living conditions. As it happens the growth of corralline algae is directly related to amount of sun in receives. For example, if it is very sunny the algae will have larger growth rings, if it is not sunny the algae will have very small growth rings. Furthermore, when the algae is growing it preserves the Mg/Ca ratio of the seawater it is growing in inside its calcite skeleton. However, if the algae is not growing the recording of Mg/Ca ratios stops. So, if there is no sea ice the algae makes thicker growth rings and has a high Mg/Ca ratio, when there is sea ice covering the area the algae is growing in the growth rings are small and the Mg/Ca ratios are low. The fact that coralline algae has two proxies that can tell us about sea ice conditions makes it an excellent tool for looking into the past to see ice coverage. It is a great double whammy!!

Fig. S2. Analytical reproducibility. Annual cycles of Mg/Ca ratios measured by electron microprobe (A) on two parallel transects along main axis of growth on uppermost 5 mm of sample Ki2 (B). Microscope image of polished sample shows ∼80 annual growth increments. Cycle shapes in A are characteristic of break in growth during sea-ice season, with narrow downward pointing peak. Summer portions of cycles instead are smooth, reflecting summer light and temperature cycle patterns following spring breakup of sea ice. Note similarity of winter Mg/Ca ratios reflecting constant seawater temperature under sea ice. Sample spacing, 15 μm (average annual growth of Ki2 = 172 μm) results in average resolution of ∼11.5 samples per year. Mg/Ca ratios are based on atom percent of Mg and Ca. Photo and caption used in accordance with PNAS guidelines for educational purposes only.
So, based on the fact that the size of the growth rings and Mg/Ca ratios in the growth rings of Clathromorphum tell us about sea ice coverage the authors set out to sample Clathromorphum from a variety of locations around Northern Canada. What they found is pictured in the figure below, but I’ll cover the highlights.
1. The record of sea ice coverage obtained from the coralline algae proxy agrees well with known sea ice coverage data from the 1970’s onwards.
2. The algal proxy shows that sea ice coverage has been declining steadily for the past 150 years, the latter part of which agrees with actual observations of sea ice coverage.
3. A 646 year record shows that sea ice coverage was high between 1530 and 1860, which corresponds to the Little Ice Age.
4. The results from this proxy agree well with those from other proxies such as ice cores and anthropological evidence for high sea ice coverage during the Little Ice Age.

Fig. 3. Algal proxy record (red) compared with observational (blue) and proxy (green) data (see Methods for detail on records). Gray lines represent 5-y moving average and colored lines, 15-y low-pass filtered (Savitzky–Goley) annual data. Algal proxy time series plotted on inverted scale to indicate declining ice cover. Light gray bars show periods of positive algal anomalies, reflected by negative ice anomalies in observational records, and positive δ18O ice core excursions. Linear trends for all time series are plotted from 1850 onwards. All individual algal specimens yield similar trends (Fig. S3). RC indicates position of radiocarbon analyses. Values are 2σ calibrated results (Table S1); all values fall well within age model derived from growth increment counting.
So there it is. Coralline algae is a great new proxy for sea ice coverage. I am left wondering a few things about the use of this proxy that hopefully the authors will address in future work. The foremost question in my mind is: could this be used as a proxy for sea ice coverage into the distant past? We find a lot of coralline algae in the fossil record so perhaps if the paleo-latitude was known, these preserved specimens could be used to interpret sea ice conditions thousands or even millions of year ago? What do you think? I’m not sure how well Mg/Ca ratios are preserved, but the growth rings certainly are….
Halfar J., Adey W.H., Kronz A., Hetzinger S., Edinger E. & Fitzhugh W.W. (2013). Arctic sea-ice decline archived by multicentury annual-resolution record from crustose coralline algal proxy, Proceedings of the National Academy of Sciences, 110 (49) 19737-19741. DOI: 10.1073/pnas.1313775110
The article that first called my attention to this paper.