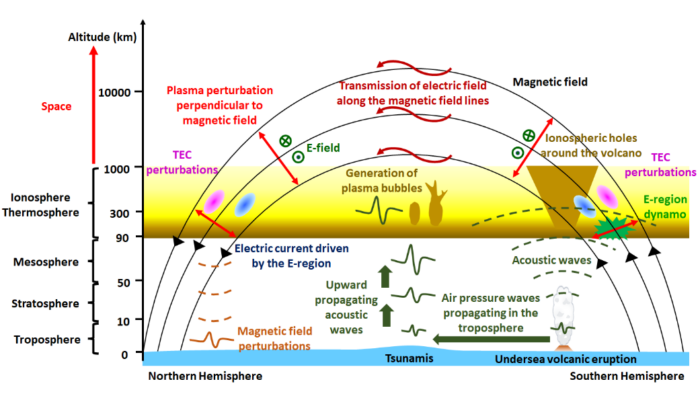
1. Introduction
Severe natural phenomena of geophysical, hydrological, and meteorological origins cause natural hazards that negatively impact human activity or the environment. Many people living on the earth suffer from natural hazards every year. Although we can predict some natural hazards (e.g., typhoons) in near real-time, forecasts of the geophysical natural hazards such as large earthquakes, volcanic eruptions, and tsunamis are challenging issues. Therefore, we have to rapidly and timely observe the key signals of natural geophysical hazards to reduce human loss. Among these geophysical hazards, the Hunga Tonga–Hunga Ha’apai (HTHH) undersea volcano’s explosive eruption occurred at 04:15 UT on 15 January 2022. This eruption is one of the most explosive events in the modern era and caused global atmospheric and ionospheric disturbances in a broad atmospheric region from the troposphere to the ionosphere (Wright et al., 2022) in addition to a tsunami in the Pacific Ocean generated by an air pressure wave (Kubota et al., 2022). The HTHH volcanic eruption provides a good opportunity to promote interdisciplinary studies of coupling processes in lithosphere-atmosphere-ionosphere to reduce disaster risks with different field datasets.
The ionosphere is a region having charged particles produced through partial ionization of the upper atmosphere above 60 km altitude due to solar extreme ultraviolet (EUV) radiation and energetic particle precipitation coming from interplanetary space and the magnetosphere. The spatial distribution of plasma density in the ionosphere depends strongly on latitude, longitude, altitude, and local time. Based on the height profile of electron density, the ionosphere is classified into several ionospheric regions: D (60–90 km), E (90–150 km), and F (150–1000 km). The peak height of the electron density is typically ~250–400 km. The ionospheric electron density profile is determined by the ionization of neutral particles, their recombination processes, dynamics, and electrodynamics in the upper atmosphere and ionosphere. Further, the ionosphere varies through solar/geomagnetic activities and upward propagating atmospheric waves originating from lower atmospheric disturbances (Kelley, 2009).
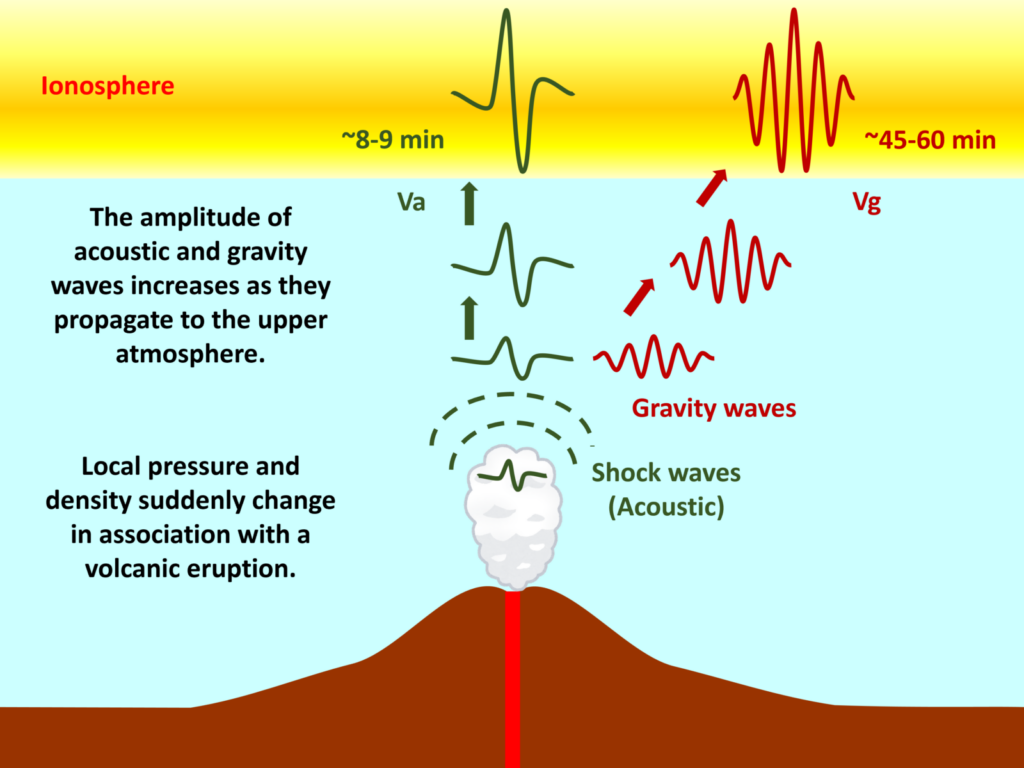
Figure 1. Schematic view of the generation process of atmospheric and ionospheric disturbances associated with a volcanic eruption. After the onset of a volcanic eruption, acoustic and gravity waves are generated by local pressure and density variations around the epicenter of the eruption. The amplitude grows with increasing altitude because the background atmospheric density decreases with increasing altitude. The acoustic waves propagating at the sound velocity (Va) arrive in the ionosphere within ~8–9 minutes after the onset of a volcanic eruption. Because the propagation velocity of the gravity waves is much slower than that of the acoustic waves, the gravity waves reach the ionosphere within ~45–60 minutes after the eruption. (Shinbori et al., 2023b)
Because the ions in the E region frequently collide with the neutral upper atmosphere and move across earth’s magnetic field lines, this process generates an electric current in the ionosphere and a dynamo electric field through momentum transfer by ion-neutral collisions (e.g., Maeda and Kato, 1966; Richmond, 1979). The dynamo electric field is instantaneously transmitted along magnetic field lines to the F region of the ionosphere and triggers the plasma density disturbances associated with the drift of ionospheric plasma (e.g., Yamazaki and Maute, 2017; Shinbori et al., 2022). The spatial inhomogeneity of ionospheric conductivity due to the plasma density perturbation creates a polarization electric field to keep the current continuity. Oscillations of the neutral atmosphere or polarization electric fields can generate traveling ionospheric disturbances (TIDs). Some of the TIDs can subsequently initiate equatorial plasma bubbles (EPBs). The origin of the neutral particle oscillations is upward propagating atmospheric waves (acoustic and gravity waves) generated by lower atmospheric disturbances such as typhoons, earthquakes, and volcanic eruptions. The transit time of acoustic and gravity waves to reach the height of the peak electron density in the ionosphere is estimated as 8–9 and 45–60 minutes, respectively (Astafyeva, 2019). Therefore, we can detect ionospheric disturbances related to the geophysical natural hazard events based on global ionospheric observations (e.g., Global Navigation Satellite System (GNSS)-total electron content (TEC) technique) approximately 10 minutes after some geophysical natural hazard events occurred as shown in Figure 1.
2. Observations of ionospheric disturbances associated with the HTHH volcanic eruption
Taking advantage of the dense GNSS receiver network in New Zealand, Australia, and Japan, Lin et al. (2022) showed that TIDs appeared over Japan approximately 3 hours before the initial arrival of an air pressure wave in the troposphere launched by the HTHH volcano. The waveform of TIDs over Japan is almost consistent with that over Australia, and the wave structure of TIDs has a magnetic conjugate feature. Because the TIDs appeared over Australia a few hours before the tsunami reached the Australian coasts, Han et al. (2023) pointed out the possibility of the development of tsunami warning system by monitoring the ionosphere with a GPS-TEC technique. Lin et al. (2022) interpreted that the TIDs are driven by electromagnetic coupling through the magnetic field lines in both hemispheres. As shown in Figure 2, Shinbori et al. (2022) demonstrated that the TIDs appeared over Japan are triggered by the electric field perturbations propagating along the magnetic field lines from the Southern Hemisphere, based on an integrated analysis of global GNSS-TEC and ionospheric plasma flow data obtained from the super dual auroral radar network (SuperDARN) Hokkaido pair of radars (Nishitani et al., 2019). This finding implies that the lower atmospheric disturbances caused by the HTHH volcanic eruption could generate the TIDs with magnetic conjugate property even in the daytime, which had not been previously reported in the literature. As shown in panel (g) in Figure 2, an enhancement of the northward plasma flow was observed in the nighttime Japanese sector associated with the arrival of the air pressure wave. In this case, the TEC perturbation related to the plasma flow variation was not detected in the Southern Hemisphere. Shinbori et al. (2023b) interpreted the reason as a difference in ionospheric conductivity around the source region between the daytime and nighttime conditions.
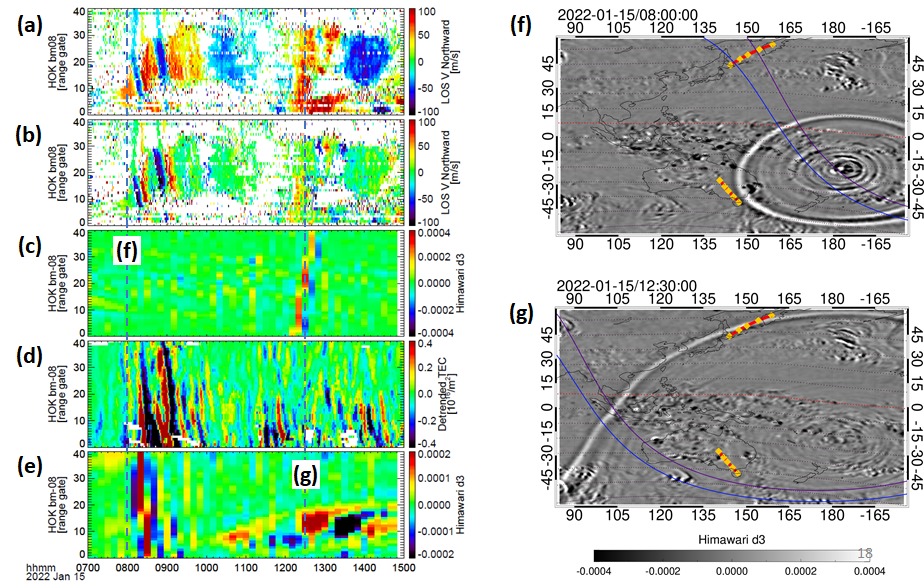
Figure 2. Range-time plots of (a) northward plasma flow, (b) 15-min high-pass filtered northward plasma flow, (c) and (e) temperature deviation, and (d) detrended TEC. The first three panels show the data along the beam-8 direction of the SuperDARN radar in the northern hemisphere, indicated by the red line in panels (f) and (g), while the rest panels show the data along the magnetically conjugated beam-8 direction in the southern hemisphere. Panels (f) and (g) show the two-dimensional map of temperature deviation at 08:00 and 12:30 UT on 15 January 2022. These times are represented by the vertical dashed lines in the left panel. The blue and red curves indicate the sunset terminator at an altitude of 105 and 300 km, respectively. (Shinbori et al., 2023b)
EPB is one of the plasma density irregularities in the ionosphere with a sharp plasma density depletion frequently observed in the nighttime equatorial and low-latitude regions. The EPB is generated by the Rayleigh-Taylor (R-T) instability at the bottom side of the F-region. It has been thought that atmospheric waves originating from lower atmospheric phenomena become a seed of the R-T instability. The plasma density irregularity related to the EPB causes signal fading, scintillation, and loss-of-lock in satellite-based communication and navigation systems. For the first time, Aa et al. (2022) identified EPBs with small-scale plasma density irregularities over the Asia-Oceania region after the HTHH volcanic eruption based on ground-based GNSS-TEC and Swarm/ICON satellite observation data analysis. Sun et al. (2022) also found the EPB occurrence at midlatitudes (up to ~35o in geographic latitude) over China after the passage of the sunset terminator at an E-region altitude (100 km). Further, Shinbori et al. (2023a) showed that the EPB observed over 100–150o E extended to ~30o N in geomagnetic latitude after the arrival of the air pressure waves propagating in the troposphere (Figure 3a). The apex altitude is estimated as ~3000 km.
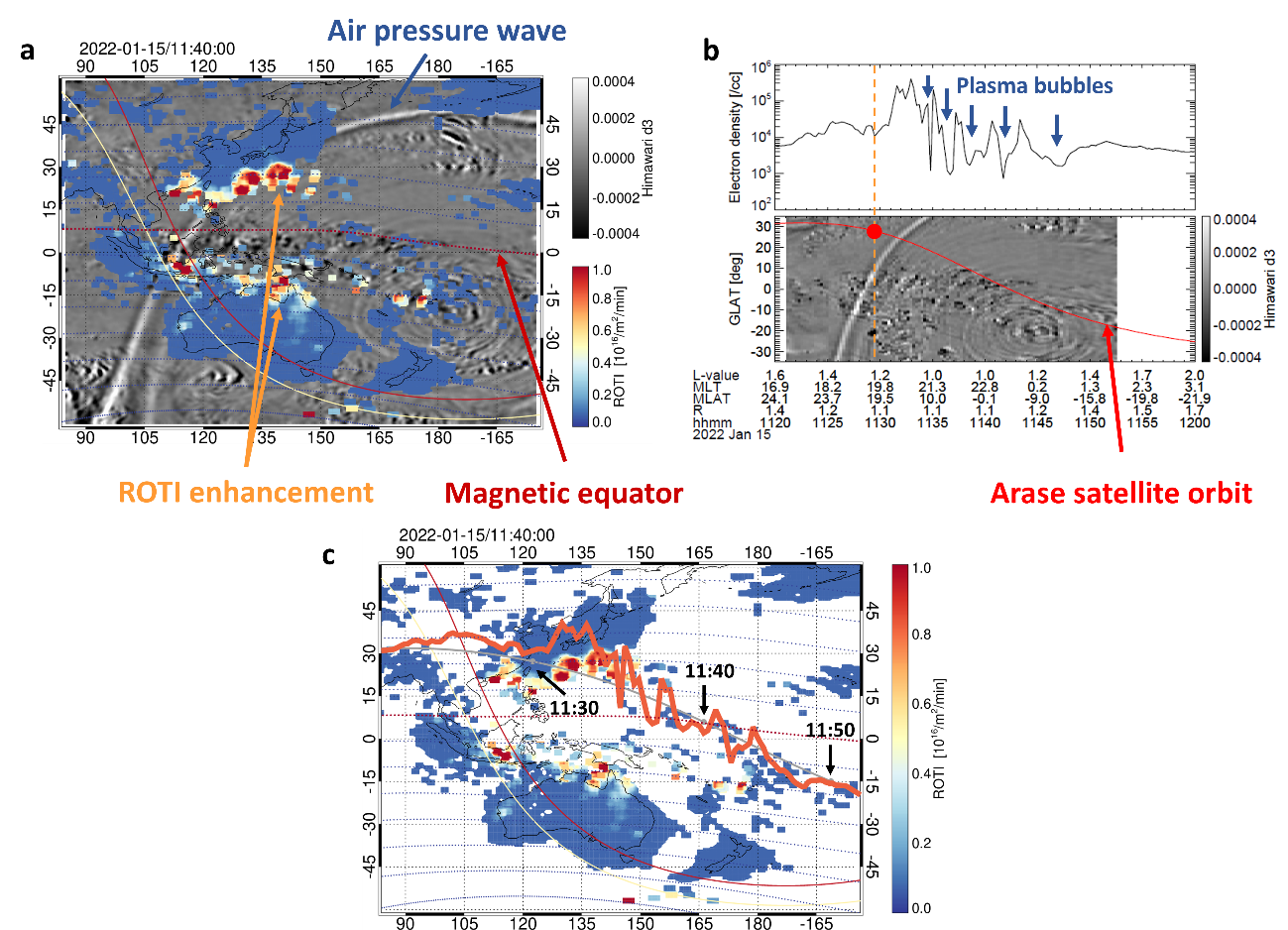
Figure 3. Observation of the EPB and air pressure wave between 11:20 to 12:00 UT on 15 January 2022. (a) Two-dimensional map of ROTI and the Himawari-8 temperature deviation (d3) at 11:40 UT, indicated by the color and gray scales, respectively. The yellow and red lines represent the 105 and 300 km sunset terminators. The horizontal dashed curves show the geomagnetic latitude every 10o. (b) Electron density observed by the Arase satellite and geographic latitude-time plot of Himawari-8 d3 data along the geographic longitude of the Arase satellite location. (c) Two-dimensional map of ROTI with the electron density along the Arase satellite path in the same period as in Figure 3(b). The smoothed gray curve is the Arase satellite orbit, while the thick orange line around the smoothed curve is the electron density variation (Shinbori et al., 2023a).
To confirm the EPB occurrence at an altitude of the ionosphere, Shinbori et al. (2023a) analyzed the electron density data derived from in-situ plasma waves (upper limit frequency of upper hybrid resonance (UHR) waves) observed by the Arase satellite (Miyoshi et al., 2018). Figure 3b shows several electron density depletions of one or two orders of magnitude after the rapid increase associated with the arrival of the air pressure waves. The occurrence region of electron density depletions is almost consistent with the enhanced Rate of TEC Index (ROTI) region (Figure 3c). In this case, the Arase satellite flew into the ionosphere of the evening sector after the sunset terminator, and the EPB was detected from 400 km (ionosphere) to at least 2000 km. Such super plasma bubbles could be caused by the combination of volcano-induced atmospheric perturbations and strong pre-reversal enhancement in the evening ionosphere.
3. What did we learn through the HTHH volcanic eruption?
The explosive eruption of the HTHH volcano generated acoustic waves, atmospheric gravity waves, strong shock waves, and air pressure waves (Lamb waves) to propagate in the troposphere. These atmospheric waves caused tsunamis and ionospheric disturbances detected by ground-based instruments worldwide. Such a global-scale atmospheric and ionospheric response to a large volcanic eruption had not yet been observed since the 1980s when the global observation of the Earth’s atmosphere and ionosphere started. Therefore, the HTHH volcanic eruption provides a unique opportunity to promote interdisciplinary studies of coupling processes in lithosphere-atmosphere-ionosphere with ground-based and satellite observations and modeling.
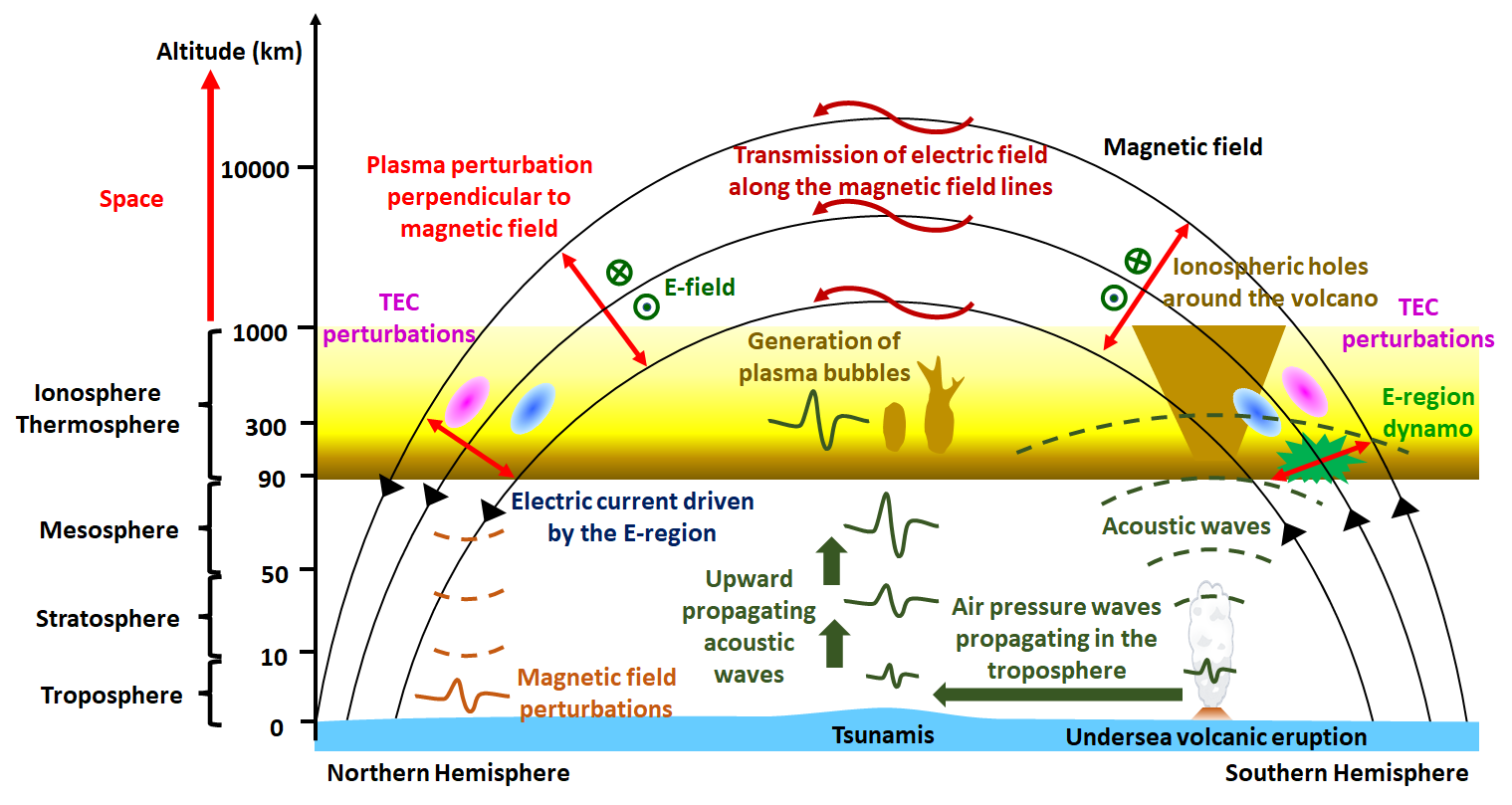
Figure 4. Schematic view of the physical processes of atmospheric and ionospheric disturbances observed after the explosive eruption of the HTHH volcano. The vertical axis in the left panel indicates the altitude and atmospheric layers. (Shinbori et al., 2003b)
Our ground-based and satellite observational results indicate that the explosive eruption of the HTHH volcano had a significant impact on space, the ionosphere, and the atmosphere. Figure 4 summarizes a schematic view of the physical processes of atmospheric and ionospheric disturbances triggered by the HTHH volcanic eruption. Studies on the coupling processes in the atmosphere-ionosphere system from the HTHH volcanic eruption highlight the need to consider connections to space at altitudes higher than the ionosphere during cataclysmic natural events. Therefore, in space weather research, we should also account for ground-based natural phenomena when examining space environmental changes related to solar activity and geomagnetic disturbances. On the other hand, recent studies on lithosphere-atmosphere-ionosphere coupling through the HTHH volcanic eruption provide new insights into heliospheric science, helping us understand space environmental changes triggered by volcanic eruptions. To advance such frontier studies, strong collaboration across various geoscience fields such as volcanology, meteorology, upper atmospheric physics, and plasma physics is essential.
References
Aa, E., Zhang, S.-R., Erickson, P. J., Vierinen, J., Coster, A. J., Goncharenko, L. P., Spicher, A., and Rideout W. (2022), Significant ionospheric hole and equatorial plasma bubbles after the 2022 Tonga volcano eruption, Space Weather, 20, e2022SW003101, doi:10.1029/2022SW003101.
Astafyeva, E. (2019) Ionospheric detection of natural hazards, Rev. Geophys., 57, 1265-1288, doi:10.1029/2019RG000668.
Han, S.-C., McClusky, S., Mikesell, T. D., Rolland, L., Okal, E., and Benson, C. (2023) CubeSat GPS observation of traveling ionospheric disturbances after the 2022 Hunga-Tonga Hunga-Ha’apai volcanic eruption and its potential use for tsunami warning, Earth, Space Science, 10, e2022EA002586, doi:10.1029/2022EA002586.
Kelley, M. (2009) The Earth’s ionosphere: Plasma physics and electrodynamics, (2nd edition), San Diego, California Academic Press.
Kubota, T., Saito, T., and Nishida, K. (2022) Global fast-traveling tsunamis driven by atmospheric Lamb waves on the 2022 Tonga eruption, Science, 377, 91-94, doi:10.1126/science.abo4364.
Liu, L., Morton, Y. J., Cheng, P.-H., Amores, A., Wright, C. J., and Hoffmann, L. (2023) Concentric traveling ionospheric disturbances (CTIDs) triggered by the 2022 Tonga volcanic eruption, J. Geophys. Res., 128, e2022JA030656, doi:10.1029/2022JA030656.
Miyoshi, Y., Shinohara, I., Takashima, T., Asamura, K., Higashio, N., Mitani, T., Kasahara, S., Yokota, S., Kazama, Y., Wang, S.-Y., Tam, S. W. Y., Ho, P. T. P., Kasahara, Y., Kasaba, Y., Yagitani, S., Matsuoka, A., Kojima, H., Katoh, Y., Shiokawa, K., and Seki, K. (2018a) Geospace exploration project ERG, Earth Planets Space, 70, 101, doi:10.1186/s40623-018-0862-0.
Nishitani, N., Ruohoniemi, J. M., Lester, M., Baker, J. B. H., Koustov, A. V., Shepherd, S. G., Chisham, G., Hori, T., Thomas, E. G., Makarevich, R. A., Marchaudon, A., Ponomarenko, P., Wild, J. A., Milan, S. E., Bristow, W. A., Devlin, J., Miller, E., Greenwald, R. A., Ogawa, T., and Kikuchi, T. (2019) Review of the accomplishments of mid-latitude Super Dual Auroral Radar Network (SuperDARN) HF radars, Progress in Earth and Planetary Science, 6, 27, doi:10.1186/s40645-019-0270-5.
Shinbori, A., Sori, T., Otsuka, Y., Nishioka, M., Perwitasari, S., Tsuda, T., Kumamoto, A., Tsuchiya, F., Matsuda, S., Kasahara, Y., Matsuoka, A., Nakamura, S., Miyoshi, Y., and Shinohara, I. (2023a) Generation of equatorial plasma bubble after the 2022 Tonga volcanic eruption, Sci. Rep., 13, doi:10.1038/s41598-023-33603-3.
Shinbori, A., Otsuka, Y., Sori, T. et al. New aspects of the upper atmospheric disturbances caused by the explosive eruption of the 2022 Hunga Tonga–Hunga Ha’apai volcano. Earth Planets Space 75, 175 (2023b). https://doi.org/10.1186/s40623-023-01930-4
Shinbori, A., Otsuka, Y., Sori, T., Nishioka, M., Perwitasari, S., Tsuda, T., and Nishitani, N. (2022) Electromagnetic conjugacy of ionospheric disturbances after the 2022 Hunga Tonga-Hunga Ha’apai volcanic eruption as seen in GNSS-TEC and SuperDARN Hokkaido pair of radars observations. Earth, Planets Space, 74, 106, doi:10.1186/s40623-022-01665-8.
Sun, W., Kuriakose, A. K., Li, G., Li, Y., Zhao, X., Hu, L., Yang, S., Xie, H., Li, Y., Ning, B., and Liu, L. (2022) Unseasonal super ionospheric plasma bubble and scintillations seeded by the 2022 Tonga volcano eruption related perturbations, J. Space Weather Space Clim., 12, 25, doi:10.1051/swsc/2022024.
Yamazaki, Y., and Maute, A. (2017) Sq and EEJ-A Review on the Daily Variation of the Geomagnetic Field Caused by Ionospheric Dynamo Currents, Space Sci. Rev., 206, 299-405, doi:10.1007/s11214-016-0282-z.
Wright, C. J., Hindley, N. P., Alexander, M. J., Barlow, M., Hoffmann, L., Mitchell, C. N., Prata, F., Bouillon, M., Carstens, J., Clerbaux, C., Osprey, S. M., Powell, N., Randall, C. E., and Yue J. (2022) Surface-to-space atmospheric waves from Hunga Tonga–Hunga Ha’apai eruption, Nature, 609, 741-746, doi:10.1038/s41586-022-05012-5.