To understand Earth’s changing climate, scientists often examine how the climate has varied in the past, by studying geological records. These records allow us to reconstruct past climates and help us predict planet’s responses to different climate forcings. In this context, it has long been thought that past ice ages on Earth were relatively dry, whereas the warm periods between ice ages we ...[Read More]
Misinterpretation of glacial aridity in subtropical environments in the Southern Hemisphere
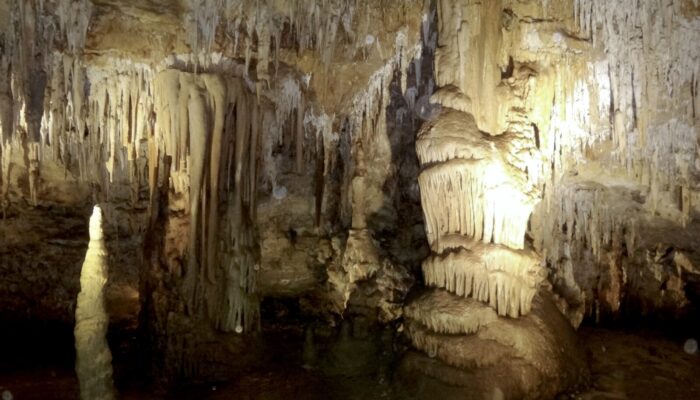