What are the most interesting, cutting-edge and compelling research topics within the scientific areas represented in the EGU divisions? Ground-breaking and innovative research features yearly at our annual General Assembly, but what are the overarching ideas and big research questions that still remain unanswered? We spoke to some of our division presidents and canvased their thoughts on what the ...[Read More]
Geoscience hot topics – Part II: the Earth as it is now and what its future looks like
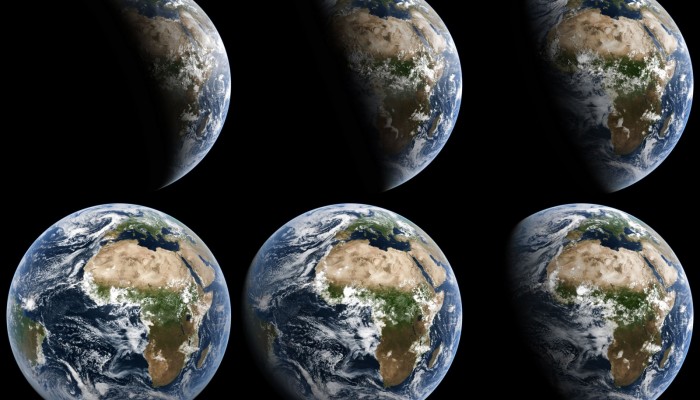