Why are we climate scientists? For us, there is a number of reasons: we feel a strong bond to nature, we like to solve puzzles and we want to understand the mechanisms of what we see every day. And – even if it only manifests at the end of a causal chain – we want to contribute to a just and livable world via working in climate science. Thus, due to distant and abstract state funding ...[Read More]
Modelling the heat mitigation effects of blue roofs and green roofs to assess climate change adaptation potentials in dense urban environments
Urban areas often show higher temperatures than their surrounding rural areas, especially during heat events. This phenomenon is called the Urban Heat Island (UHI) effect. The magnitude of the UHI effect is expressed by the absolute temperature difference between the rural and the urban area and can reach more than 10 °C. During past decades, the magnitude of the UHI effect has intensified in many ...[Read More]
Misinterpretation of glacial aridity in subtropical environments in the Southern Hemisphere
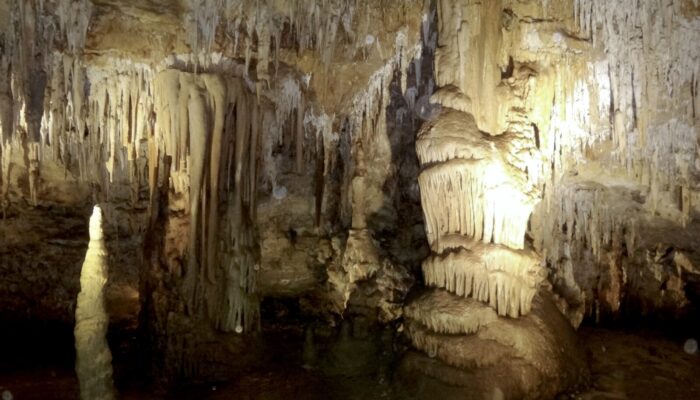
To understand Earth’s changing climate, scientists often examine how the climate has varied in the past, by studying geological records. These records allow us to reconstruct past climates and help us predict planet’s responses to different climate forcings. In this context, it has long been thought that past ice ages on Earth were relatively dry, whereas the warm periods between ice ages we ...[Read More]
Spotlighting the Climate Division’s sessions for EGU24
Dear community of climate enthusiasts and EGU lovers, We know that being part of the EGU is not just about staying in the loop with the latest geoscience works – especially when it comes to our all-time favorite realm of sciences: climate sciences 🤩. It is also an amazing opportunity to spark exciting collaborations and expand your network with scientists from all over Europe and the world. EGU is ...[Read More]